SRTM (Shuttle Radar Topography Mission)
EO
NASA
Mission complete
Land
Quick facts
Overview
Mission type | EO |
Agency | NASA |
Mission status | Mission complete |
Launch date | 11 Feb 2000 |
End of life date | 22 Feb 2000 |
Measurement domain | Land |
Measurement category | Multi-purpose imagery (land), Landscape topography |
Measurement detailed | Land surface imagery, Land cover, Land surface topography |
Instruments | Advanced Scatterometer, GPS receiver |
Instrument type | Other, Scatterometers, Data collection, Imaging microwave radars |
CEOS EO Handbook | See SRTM (Shuttle Radar Topography Mission) summary |
SRTM (Shuttle Radar Topography Mission)
Mission overview Launch SRTM Mission Hardware Sensor Complement Ground Operations SRTM Observation Coverage Ground Segment Calibration Campaigns References
SRTM is a joint Shuttle mission (international project) between NASA, DoD/NGA (National Geospatial-Intelligence Agency - formerly NIMA), DLR (German Aerospace Center - Deutsches Zentrum für Luft- und Raumfahrt), and ASI (Agenzia Spaziale Italiana). Funding for SRTM is from NIMA [Note: As of Nov. 2003, NIMA (National Intelligence Mapping Agency) changed its name to NGA (National Geospatial-Intelligence Agency)], while NASA is supplying the SIR-C hardware, the Shuttle launch, ground systems, mission operations and data processing support. DLR and ASI are supplying the X-SAR hardware, ground systems, mission operations and data processing support.
SRTM represents a reflight of the basic SIR-C/X-SAR payload instruments on the SRL-1 (STS-59, April 9 - 20, 1994) and SRL-2 (STS-68, Sept, 30 to Oct. 11, 1994) missions. However, the SRTM configuration considers only two independent SAR systems, one in C-band (JPL instrument), the other in X-band (DLR/ASI). Both SAR systems operate with the main antenna of each instrument located in the open cargo bay of the Shuttle, and a second receive antenna mounted on a deployable outboard mast, respectively. The configuration (a bistatic system) for both radar systems represents a single-pass interferometer, also referred to as IFSAR, with a baseline of 60 m (separation of the two antenna systems). The mission is managed by NASA/JPL. 1) 2) 3) 4) 5) 6) 7) 8) 9)
The SRTM mission is in fact the first fixed baseline single-pass spaceborne InSAR(InterferometricSynthetic Aperture Radar) technology system with wide-swath ScanSAR and dual-frequency (C-band and X-band) coverage as well as with dual-polarization capability. As such, the mission represents one of the most significant mapping surveys from space of our planet so far undertaken.
The mission objective is to obtain single-pass interferometric SAR imagery to be used for DEM (Digital Elevation Model, also referred to as DTM = Digital Terrain Model) generation, i.e. topographic maps. Coverage of the Earth's land surfaces is provided between the latitudes of -54º and +60º, representing nearly 80% of the land masses.
The digital topographic map production objective (C-RADAR) calls for requirements which meet ITHD-2 (Interferometric Terrain Height Data -2) specifications, i.e., a spatial pixel (30 m x 30 m) posting with a 16 m absolute vertical linear accuracy and 20 m absolute horizontal radial accuracy at 90%. - Applications: the topographic data are being used for such studies as: flooding, erosion, landslide hazards, earthquakes, weather forecasts, climate zones, etc.
Mission Overview
SRTM employed two synthetic aperture radars, a C-band system (5.6 cm; C-RADAR) and an X-band system (3.1 cm; X-RADAR). NASA's Jet Propulsion Laboratory (JPL) was responsible for C-RADAR. The DLR with EADS Astrium (formerly Dornier Satellitensysteme, GmbH), its contractor for the X-band space segment, was responsible for X-RADAR. The operational goal of C-RADAR was to generate contiguous mapping coverage as called for by the mission objectives. X-RADAR generated data along discrete swaths 50 km wide. These swaths offered nearly contiguous coverage at higher latitudes.
X-RADAR was included as an experimental demonstration. As it did not employ ScanSAR, the X-band radar had a slightly higher resolution and better signal to noise ratio (SNR) than the C-band system. Thus it could be used as an independent data set to help resolve problems in C-RADAR processing and quality control.
SRTM was an example of engineering at its best; it marked a milestone in the field of remote sensing. In the span of 7 years, the project evolved from concept to final data product, with 4 years of flight segment development, 10 days of observations, and one additional year of ground processor development. This was capped by 9 months of data production.
Launch
The SRTM payload was launched on Feb. 11, 2000 onboard Space Shuttle Endeavour (STS-99). The 11-day SRTM Shuttle mission took place in the period Feb. 11-22, 2000. The total mass of the SRTM payload was 14,500 kg. 10)
Orbit
Circular exact repeat orbit, altitude = 233 km, inclination = 57º. The Shuttle flew upside down and tail forward for simultaneous radar observations.
The look angle for the C-RADAR antenna system is 45º, for the X-RADAR antenna system = 52º. A nominal data collection period of 80 hours is assigned for both radars. Known orbit with respect to WGS 84 ellipsoid.

SRTM Mission Hardware
The SRTM architecture was based on the SRL SIR-C/X-SAR instruments, modified and augmented to enable single-pass interferometric operations. The resulting new system consisted of four principal subsystems: C-RADAR, X-RADAR, AMS (Antenna/Mechanical System), and the AODA (Attitude and Orbit Determination Avionics).

An important addition to the original SIR-C/X-SAR mission hardware was the AODA capability. This system was required to obtain data on the length and orientation of the mast and the location and orientation of the Shuttle in earth-centered inertial coordinates. These factors are critical to the creation of an accurate digital topographic map automatically without ground control points. The on-board AODA (Attitude and Orbit Determination Avionics) consisted of a suite of sensors (target tracker, a new GPS receiver system configuration, called BlackJack for orbit and attitude determination, distance meters, inertial reference units, and star tracker) to collect attitude and position information. The AODA data rate is 8.192 kbit/s. The baseline knowledge of the two inboard and outboard antenna pairs is known within 3mm, the angular alignment knowledge is better than 9 arcsec. 11) 12) 13)
The primary function of the AODA system was to provide a post-flight time history of the interferometric baseline for use in the topographic reconstruction processing. For SRTM, AODA was required to provide estimation of the interferometric baseline length, attitude, and position to an accuracy of 2 mm, 9 arcsec, and 1 m (at 90%, or 1.6σ, confidence level), respectively. These requirements had to be satisfied throughout the entire mission whenever the radars were collecting data, at a rate better than 0.25 Hz. During instrument development, however, AODA took on the additional requirement to support mission operations, including verification of in-flight mast deployment, antenna alignment, and Shuttle attitude control optimization.
To get the most accurate measure of the mast length, SRTM is using a set of rangefinders, called EDM (Electronic Distance Measurement) units. The rangefinders work by bouncing a beam of light off a special corner-cube reflector on the outboard antenna and measuring the time to determine the distance.

Legend to Figure 3: SRTM hardware in Shuttle payload bay. The upper diagram shows the stowed configuration. At lower left is the deployed OASYS (Outboard Antenna System). Lower right shows details of the ASP (AODA Support Panel).
Payload Mast
The mast used on the SRTM mission is the ADAM (Able Deployable Articulated Mast) built by the AEC-Able Engineering Company, Inc. of Goleta, CA. The mast is a truss structure that consists of 87 cubical sections (referred to as bays) with a total mass of 360 kg. Unique latches on the diagonal members of the truss allowed the mechanism to deploy bay-by-bay out of the mast canister to a length of 60 m. The canister housed the mast during launch and landing, and it also deployed and retracted the mast. 14)
The performance of the instrument, the mast, the alignment of the inboard and outboard antennas, the function of the recorders of the AODA and the performance of the orbiter and its subsystem were so good, that some features of the payload system had not to be used at all. Due to the low dynamic motion of the mast the auto beam tracker signals were not needed to match the inboard and outboard antenna beams with variable pointing of the outboard antenna. Only a slight static misalignment was compensated with electronic beam steering. 15) 16) 17)


The stiff and rigid mast (or boom) can experience length variations of up to 10 mm (thermal distortion) and bending/twist up to 0.1º during radar data acquisition. The total mast plus outboard antenna system has a mass of 1700 kg. Since the long boom tends to drift into a gravity-gradient attitude (where one end of the S/C points toward Earth), this has to be counteracted by a thruster system mounted on the boom structure.
The outboard antenna structure was connected to the end of the mast featuring two receive-only antennas, C-Band and X-Band, two GPS antennas, LED (Light Emitting Diode) targets, and a corner-cube reflector. The GPS antennas were used to gather accurate position information for the Space Shuttle. The LED targets were used by the target tracker on the AODA to measure the position of the outboard antenna relative to the main antenna. The corner-cube reflector was used by the electronic distance measurement unit on the AODA to measure the length of the mast to within 3 mm.


Note: The Shuttle flew upside down and tail forward for simultaneous radar observations.
Sensor Complement
The sensor complement consists of modified SIR-C/X-SAR instruments (plus add-on structures) flown on SRL-1 and SRL-2 in April and October 1994, respectively.
Note: While the L-band radar/antenna is flown as part of the overall structure, there is no interferometric L-band capability due to the large antenna dimensions needed at the outboard location. The L-band radar is not being operated, however, some of its electronic capabilities are used for the C-RADAR.
The two single-pass interferometric SAR systems, namely C-RADAR and X-RADAR, are configured in such a way that each radar is operating a transmit/receive antenna system inside the Shuttle cargo bay (primary or inboard system) and a receive-only antenna system at the boom tip (secondary or outboard system). The boom structure is oriented in the cross-track direction, perpendicular to the velocity vector of the Shuttle, with tip-mounted antennas, forming a parallel antenna system with the inboard system. Single-pass interferometry observation requires a beam overlap geometry of the primary and secondary antenna systems. This implies alignment measures for each antenna system to compensate for possible misalignments during the mission.
• The X-RADAR system provides a two-axis alignment capability. The inboard antenna can be steered mechanically (tilt of ±1.5º) in the cross-range direction to fine-tune the footprint of the outboard antenna. In addition, the outboard antenna can be steered electronically (±1º) in the azimuth direction to match the footprint of the inboard antenna. 18)

Legend to Figure 8: The ellipsoids represent the C-Band scan swathes, the X-SAR swath is located around 54.5º look angle.

• The phased array antenna system of C-RADAR provides 2-D electronic steering of the inboard and outboard systems for beam alignment. Four C-band subswaths of the inboard system are generated sequentially in ScanSAR mode, where two beams are forming an instantaneous ScanSAR pair, each pair producing a subswath with V and one with H polarization. Two parallel transmit/receiver channels are employed for this purpose, one is of the C-band system, the other channel is “borrowed” from the otherwise dormant L-band system.
C-RADAR (C-band Radar Instrument)
C-RADAR (5.3 GHz, wavelength = 5.6 cm, bandwidth = 10 MHz) is provided by NASA/JPL, it offers a dual-polarization capability. The peak radiation power is 1.2 kW/polarization. In ScanSAR mode, C-RADAR employs two simultaneous beam pairs, as shown in Figure 8, which switch back and forth within the full swath width, creating a contiguous and overlapping SAR imagery pattern. The total swath width is 225 km providing a pixel size (resolution) of about 30 m (data point spacing of one arcsec).
Instrument source data are collected at 180 Mbit/s (in four channels) and stored on the on-board recorders. Instrument telemetry data at 15.12 kbit/s is downlinked continuously.
X-RADAR (X-band Radar Instrument)
X-RADAR (9.6 GHz, wavelength = 3.1 cm) is provided by DLR and ASI, it offers fixed beam (52º look angle) single polarization (VV inboard and V outboard antenna). The peak radiation power is 1.7 kW at a PRF of 1674 pulses/s. X-RADAR operates in a wide-swath mode of 50 km, the horizontal pixel resolution is 30 m x 30 m with a vertical height resolution of 6m relative and 10 m absolute. The X-SAR swath is positioned in the middle of the two outer subswaths of C-RADAR.
The instrument science data rate is 90 Mbit/s and stored on the on-board recorder. Instrument telemetry data at 9.6 kbit/s is downlinked continuously. 19) 20)
Downlink of Snapshot Data
The source data rates of both radars were higher than the Shuttle’s downlink capacity (45 Mbit/s). This required the use of onboard high-rate data tape recorders. Selected snapshots of data necessary for near-real-time performance assessment were downlinked via the Shuttle Ku-band and NASA's TDRS (Tracking and Data Relay System) link to JPL in Pasadena and to the Payload Operations Control Center (POCC) in Houston.
Parameter | L-band Antenna | C-band Antenna | X-band Antenna |
Frequency | 1.25 GHz | 5.3 GHz | 9.6 GHz |
Bandwidth |
| 10 MHz | 9.5 MHz |
Inboard antenna: |
| transmit/receive | transmit/receive |
Outboard antenna: |
| receive only | receive only |
Architecture | Active Phased Array | Slotted waveguide array | |
Phase control | 4 bit | 4 bit | N/A |
Polarization | 4 subswaths transmit/receive H,V,V,H, inboard | VV inboard antenna, | |
Polarization isolation | 25 dB | 25 dB | 39 dB |
Antenna gain | 36.4 dB | 42.7 dB | 44.5 dB |
Mechanical steering range | N/A | N/A | ±23º, fixed at 7º SRTM |
Electronic steering range | ±20º | ±20º | ±0.9º |
Elevation beamwidth | 5-16º | 5-16º | 5.5º |
Azimuth beamwidth | 1.0º | 0.25º | 0.14º (0.28º) |
Transmit pulse width |
| 34µs | 40 µs |
Look angle (adjustable off-nadir angle) | 15º - 55º | 15º - 55º | 52º, 17º - 60º possible |
Peak radiated power | 4400 W | 1.2 kW/polarization | 1.7 kW |
System noise temperature | 450 K | 550 K | 551 K |
PRF |
| 1344-1550 Hz | 1440-1674 Hz |
Swath width |
| 225 km | 50 km |
Ground resolution |
| 30 m x 30 m | 30 m x 30 m |
Height resolution relative |
| 10 m | 6 m |
Quantization |
| 8 bit (equivalent) | 6 bit I and 6 bit Q |
Data rate |
| 180 Mbit/s(4 x 45 Mbit/s) | 90 Mbit/s (2 x 45 Mbit/s) |
Both radar systems onboard, though controlled individually to be independent and to optimize their specific radar settings, have to be operated jointly at the same start and stop times. The X-SAR instrument was operated by a DLR operations team and the C-band radar system by a JPL team at the NASA Johnson Space Center Payload Operations Control Center (POCC) in Houston.
The X-SAR system had its own Space Shuttle interface for power , command, telemetry, time, orbit and attitude information and data links. All jointly used flight hardware like the mast, AODA (Attitude and Orbit Determination Avionics), high rate data Recorder Interface Controller and the DDRE (Digital Data Routing Electronics) was provided and operated by JPL (Ref. 15).
Secondary Experiment
EarthKAM (Earth Knowledge Acquired by Middle school students), a NASA sponsored education program, PI: Sally Ride, UCSD. EarthKAM aboard STS-99 took 2,715 digital photos during the SRTM through an overhead flight-deck window. The program lets students select photo targets and receive the images through the Internet. The pictures are used in classroom projects on Earth science, geography, mathematics and space science. 21)
Background: The camera program started in 1996 as KidSat on Shuttle flight STS-76. EarthKAM was flown on four flights prior to STS-99 which resulted in a combined image total of 2,018 pictures. These photographs included the Himalayas, clouds over the Pacific, volcanoes, and recent forest fires in Indonesia. - EarthKAM was taken onboard the ISS with STS-98 (Feb. 7-21, 2001) and installed on ISS as “ISS EarthKAM.” EarthKAM photographs are taken by remote operation from the ground.

Ground Operations
The SRTM ground teams, located at the POCC (Payload Operations Control Center) at NASA/JSC (Johnson Space Center) in Houston, the Customer Support Room at JSC, and the Mission Support Area (MSA) at JPL, controlled the rest of the SRTM activities. Each of the two SRTM radars was operated by a separate system centered at the POCC. Operations of C-RADAR were handled by the MOS (Mission Operations System) and those of X-RADAR by the MPOS (Mission Planning and Operations System).
The JPL MSA, networked to the POCC, was responsible for processing C-RADAR data downlinked from the Shuttle during the mission. DLR had a similar system set up in Germany. The downlink connection to JPL was routed through the NASA Tracking and Data Relay Satellite System and the White Sands Ground Terminal, while a separate high-rate data link was used to transmit processed images and measurements to JSC (Ref. 1).
The operating systems for C-RADAR and X-RADAR performed parallel and equal tasks: mission planning, instrument commanding, instrument health monitoring, and instrument and system performance analysis. The MOS consisted of six major subsystems (Figure 11):
• MPS (Mission Planning Subsystem)
• CMS (Command Management Subsystem)
• TMS (Telemetry Management Subsystem)
• PES (Performance Evaluation Subsystem)
• DMS (Data Management Subsystem)
• ATMA (AODA Telemetry Monitor/Analyzer)

Based on the mission timeline, MPS generated the C-RADAR command input file. Using the command input files produced by MPS, the CMS (Command Management Subsystem) generated time-tagged commands for uplink to C-RADAR. CMS also had the capability to generate immediate (real-time) commands. In addition, CMS received AODA block commands and formatted them for uplink to the AODA flight instruments. The TMS (Telemetry Management Subsystem) could be viewed as the CMS downlink counterpart. TMS decommutated the Orbiter operational downlink telemetry and split it into the C-RADAR, AODA, Orbiter Instrumentation, and Orbiter Systems Management data streams. The all-important AODA stream was routed to the ATMA (AODA Telemetry Monitor/Analyzer) in the POCC.
Commands were sent to the onboard instruments via MCC and the S-band link to the orbiter subsystems. Instrument telemetry was received over the same link. Lastly, the PES (Performance Evaluation Subsystem) evaluated the single-channel and double-channel (interferometric) performance of the C-RADAR instrument during the mission and, in conjunction with MPS, provided orbit-by-orbit reports of those data takes which failed to meet the mission requirements (Ref. 1).
SRTM Observation Coverage
The SRTM mission fulfilled all mission objectives. The mast was deployed to it's full length without any problems and the antenna was successfully turned to its operational position. Hence, the mapping mission started less than 12 hours after launch.
Parameter | X-RADAR | C-RADAR |
Data takes | 367 | 764 |
Coverage (total) | 112 x 106 km2 |
|
Coverage (land) | 64 x 106 km2 | 119 x 106 km2 |
Mapping time | 90.6 hours | 99.2 hours |
Tapes filled | 122 | 208 |
Energy used | 164 kWh | 736 kWh |
Playback | 49 | 104 |
Raw data acquisition | 3660 GByte | 8590 GByte |
The SRTM payload generated a total volume of about 12 TByte (equivalent to 20,000 CDs) of SAR raw data which were recorded onboard onto a tape recorder. The data storage system employed high-rate data recorders (300 cartridges each of 40 GByte capacity). The C-Radar system generated 8.6 TByte of raw data while the X-Radar system accumulated 3.66 TByte of raw data. A small portion of the SAR data were downlinked during the mission to monitor the instrument performance (also preview of some ground scenes).
Postflight calibration and analysis of the data (beyond the scope of this description) and generation of the most complete high-resolution digital topographic database of Earth - were carried out at institutions in the USA (NASA/JPL, C-band data), Germany (DLR, X-band data), and Italy (ASI, X-band data over Italy). In the US, C-band data products have been transferred to the EDC (EROS Data Center) of USGS (US Geological Survey) for civilian archive and distribution, while NGA handles the distribution for DoD.
The SRTM mission provided the following coverage statistics:
1) C-band data of the C-Radar system using ScanSAR and a swath width of 225 km
• 99.98% of the targeted land surface area was mapped at least once, representing a total size of 119.51 x 106 km2
• 94.5% of the targeted land surface area was mapped at least twice, representing a total size of 113 x 106 km2
• 49.2% of the targeted land surface area was mapped at least 3 times, representing a total size of 58.5 x 106 km2
• 4.1% of the targeted land surface area was mapped at least 4 times, representing a total size of 28.8 x 106 km2
2) The X-RADAR system covered a total area of 64 x 106 km2 in 367 data takes using a swath width of 50 km (Multitemporal coverage in some areas)
The X-band system featured only a fixed-position pointing antenna (no ScanSAR capability) leading to a partial coverage of Earth's surface. However, the X-band data provide improved relative height accuracies for its DEM products.
The major advantages of SRTM/X-SAR data compared to the ERS tandem mission InSAR data are the single-pass observation mode and the flat incidence angle. The single-pass observation mode avoids temporal decorrelation and artifacts through atmospheric delay. The incidence angle of 54º (ERS 23º) avoids layover in mountainous areas. While ERS interferometry is well understood today and is an operational method, single-pass systems like X-SAR/SRTM bring up new challenges on baseline and phase stability. 22)
As of Feb. 2005, NASA and NGA have completed Earth's most extensive global topographic map (this represents more than four years of data processing). The digital elevation maps encompass 80% of Earth's landmass. They reveal for the first time large, detailed swaths of Earth's topography previously obscured by persistent cloudiness. The data will benefit scientists, engineers, government agencies and the public with an ever-growing array of uses.
The final data release (of 3 arcsec DEMs - 90 m pixel) covers Australia and New Zealand in unprecedented uniform detail. It also covers more than 1,000 islands comprising much of Polynesia and Melanesia in the South Pacific, as well as islands in the South Indian and Atlantic oceans. The release of this data set completes the public release of all of the SRTM 3 arcsec data - permitting studies of natural hazards such as floods, volcanic eruptions, landslides, and earthquakes in remote or poorly mapped areas. In addition, the data set will serve for many uses in remote sensing as well as in other applications. 23) 24) 25) 26) 27) 28) 29) 30) 31) 32) 33)
• September 14, 2021: Northwestern Namibia is not your typical desert landscape. Valleys dissect plateaus, creating striking vistas across the hot, dry region in southwest Africa. While these valleys are extremely old—with origins dating back hundreds of millions of years—they are remarkably well preserved. 34)
- New research indicates that the valleys are ancient relics of a time long before dinosaurs walked the Earth—when Africa was close to the South Pole, still part of the supercontinent Gondwana, and covered with ice. The flow of the ice cut into the land and eventually carved out long, narrow valleys that filled with seawater and formed fjords. Somehow, these ancient fjords have avoided being erased by erosion, uplift, and other geological processes that usually level off this kind of terrain. In fact, the area is so well preserved that scientists call it a “fossil glacial landscape.”
- “One who looks at these valleys has a snapshot of what the fjords looked like 300 million years ago, except that the ice here has long disappeared,” said Pierre Dietrich, a scientist at University of Rennes and lead author of the study published in Geology.
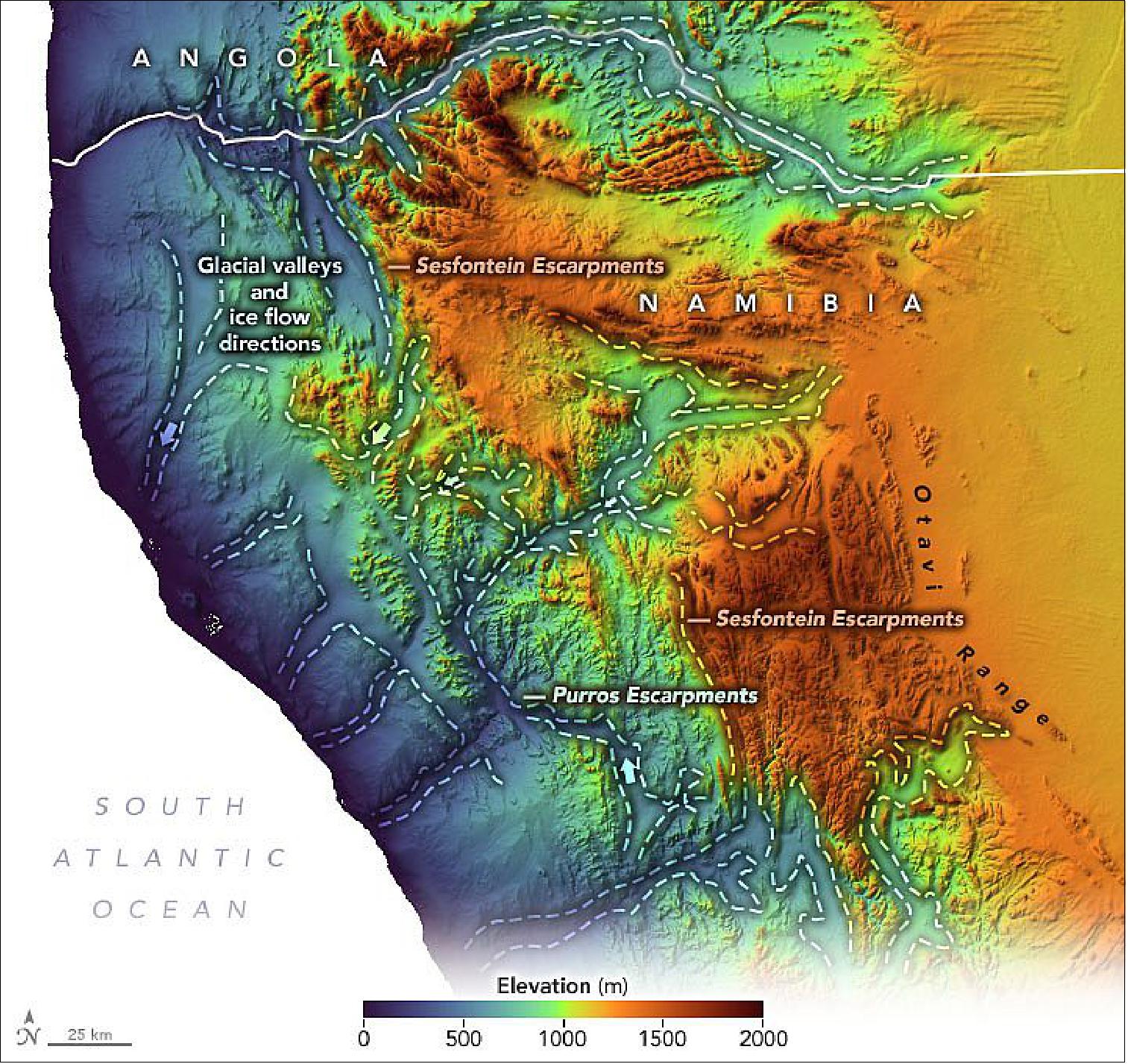
- The fossil fjords were carved around 300 million years ago during the Late Paleozoic icehouse, a period when Earth’s climate was cool and Gondwana was heavily glaciated. Today, analogs exist in the ice-carved fjords of Norway and Greenland. Although these modern examples are larger in scale, the land-ice processes are thought to be much the same.
- “It was the knowledge of the modern fjord systems that allowed us to interpret the Namibian valleys as paleofjords,” Dietrich said.

- Carved under thick flowing ice sheets that later shrank, the valleys still display evidence of their icy past. The researchers found traces of moraines—accumulations of rocks and debris moved by glacial ice—plastered on valley walls. Ice has etched scratches and grooves into the rocks (striae) and in other places sculpted them into rounded knolls (whalebacks).
- As the glaciers retreated, seawater flowed into the fjords and deposited sediments. Namibia’s fjords slowly filled with more and more sediment, which likely played an important role in preserving them. Erosional processes later began to remove the sediments, leaving behind the stronger rock of the original fjords. The photograph above, shot by Dietrich, shows a close up view of one of the region’s exhumed fossil fjords.
- “Nowadays we see the original shape of the fjords as they were when occupied by the ice,” Dietrich said. “The striae looked so fresh and pristine in Namibia that we first thought we had discovered traces of recent glaciers.”
- Sediment partly explains why the fjords have been so well preserved, but researchers still want to know how this glacial landscape escaped erosion to such an extreme degree.
- “Large-scale glacial landforms such as fjords are usually thought to be transient features, prone to be rapidly erased over geological timescales, which was obviously not the case here,” Dietrich said. “One might therefore ask: what will happen to the fjords of Greenland and Norway in a distant future?”
• As of May 2011, DLR is providing the SRTM elevation model data free of charge to the science community via the EOWEB. 35)
The X-band DEMs from the SRTM mission have a resolution of 1 arcsec, corresponding to a pixel size of 25 m at central latitudes. Each DEM covers an area of 2.5º x 2.5º and is composed of 'tiles', each measuring 15 arcmin x 15 arcmin (900 x 900 data points). They can be downloaded free of charge by registering with EOWEB. Registered users can download these datasets from an FTP server. SRTM data can be incorporated directly into digital maps or geographical information systems by means of a standardized WMS (Web Map Service).
The SRTM was not just a milestone in terms of high-precision mapping of the Earth from space; it was also the precursor to and a test for the current TanDEM-X mission, which involves two identical German radar satellites orbiting Earth in formation to record a comprehensive and even more precise DEM that is scheduled for completion in 2013.



Legend to Figure 16: The inset shows a higher resolution view of the boxed area, with a different color table to emphasize geomorphic features. The large image size is 638 km x 1113 km; the inset is 93 km x 106 km; north is up. (JPL images PIA03314 and PIA03374).
Ground Segment Calibration Campaigns
A number of ground and airborne calibration campaigns were conducted during the 11-day SRTM mission in Feb 2000 in various parts of the world. Three of them are mentioned here.
• DLR supported the following SRTM calibration activities which consisted of five phases: 36) 37)
1) Preflight concept definition phase including sensor characterization, calibration algorithm development and implementation
2) Ground campaigns during the mission
3) On-board calibration measurements during the mission
4) A 6-8 month commissioning phase for the generation of static and dynamic calibration files, and for analysis and modeling of parameter drifts with temperature and time
5) Operational calibration and validation after the commissioning phase.
DLR sites in Bavaria: For the SRTM mission, a large test site extending over almost 300 km in southern Germany was set up comprising 26 trihedral corner reflectors of different size and a number of ground receivers. All corner reflectors have been precisely surveyed using differential GPS, serving not only as references for absolute radiometric calibration, but also as absolute height reference points for DEM generation.
By using the ground receivers and the transponders, the antenna patterns of both the German/Italian X-RADAR and the NASA C-RADAR could be measured, which is required for radiometric calibration. The corner reflectors serve as reference points in the topographic data to calibrate the phase.
• The University of Michigan conducted an SRTM calibration and validation campaign. Nine sites in southern Michigan were chosen as point target calibration sites. Eight of the sites were large, open fields. The remaining site, site 4, featured the highest hill in Washtenaw County. 38) 39)
A calibration array, consisting of point and distributed targets, was deployed with the intention of determining the accuracy of InSAR-derived digital elevation maps and performing radiometric calibration of backscatter data. The array spanned one of the X-band swaths and stretched from Toledo, Ohio to Lansing, Michigan. Passive and active targets were used. The passive targets included trihedrals and tophats. Backscatter measurements at C-band and X-band of an extensive plowed field were also made for distributed target calibration. The measurements of the field were acquired by a truck-mounted radar system. The locations in latitude, longitude, and elevation of the point targets were determined using differential GPS for interferometric phase calibration.


• In 1996, the AIRSAR system of JPL embarked on a seven-week campaign to several Pacific Rim countries. This mission was jointly organized by NASA, Australia’s Office of Space Science and Application, and University of New South Wales. 40)
AIRSAR spent two weeks in Australia mapping sites in all six provinces in mainland Australia. These sites were selected by Australian scientists and mining companies, and NASA scientists. Sites in Eastern and Southeastern Australia included Condarnine, Goulbum, and Tarrawarat which were imaged to generate soil moisture maps. Mt. Fitton is an international radar calibration site rich in minerals and geologic features such as alluvial fans and sand dunes. Lake Acraman was imaged to study an ancient meteor impact crater whereas Cooper Creek was imaged to study the history of seasonal river channels in this desert region. In addition, a 500 km long transect was imaged over the Cunnamulla region just west of Brisbane to provide a valuable verification data set for the upcoming Shuttle Radar Topography Mapping (SRTM) mission.
In Northeastern Australia, Gilbert Range was imaged for hydrological studies and Lawn Hill was imaged to study a meteor impact crater. Weipa was a site selected for SRTM calibration.
The Taiwan sites were organized by the Center for Space and Remote Sensing Research Center of National Central University in Chung Li. Two large study sites were selected for land cover and coastal zone mapping using both XTI and POLSAR modes. In addition, two transects were imaged for SRTM verification. One of these transects crossed the tip of the Oluan Peninsula in southern Taiwan whereas the other transect crossed central Taiwan.
References
1) T. G. Farr, P. A. Rosen, E. Caro, R. Crippen, R. Duren, S. Hensley, M. Kobrick, M. Paller, E. Rodriguez, L. Roth, D. Seal, S. Shaffer, J. Shimada, J. Umland, M. Werner, M. Oskin, D. Burbank, D. Alsdorf, “The Shuttle Radar Topography Mission,” URL: http://www2.jpl.nasa.gov/srtm/SRTM_paper.pdf
2) M. U. Werner, “X-SAR/SRTM a Spaceborne Single Pass Interferometric SAR,” Joint workshop of ISPRS WG I/1, I/3, and I/4: Sensors and Mapping from Space, University of Hannover, Germany, Sept 29 - Oct. 2, 1997
3) http://www2.jpl.nasa.gov/srtm/mission.htm
4) R. L. Jordan, E. R. Caro, Y. Kim, Y. Shen, F. V. Stuhr, M. U. Werner., “Shuttle Radar Topography Mapper,” Proceedings of the EUROPTO Conference: Symposium on Remote Sensing, Conference on Microwave Instrumentation for Remote Sensing of the Earth II, Taormina, Italy, Sept. 24-26, 1996
5) P. Chien, “Around the World in 11 Days,” Launchspace Magazine, Vol. 3.06, Dec. 1998
6) B. Rabus, M. Eineder, A. Roth, R. Bamler, “The shuttle radar topography mission - a new class of digital elevation models acquired by Spaceborne radar,” ISPRS Journal of Photogrammetry Remote Sensing, Vol. 57, Issue 4, 2003 pp. 241-262
7) http://www2.jpl.nasa.gov/srtm/
8) http://www2.jpl.nasa.gov/srtm/srtmBibliography.html
9) http://www.shuttlepresskit.com/sts-99/payload57.htm
10) http://www.shuttlepresskit.com/STS-99/payload56.htm
11) R. Duren, E. Wong, B. Breckenridge, S. Shaffer, C. Duncan, E. Tubbs, P. Salomon, “Metrology, attitude, and orbit determination for spaceborne interferometric synthetic aperture radar,” Proceedings of SPIE, Acquisition, Tracking, and Pointing XII, Vol. 3365, 1998, pp. 51-60, URL: http://www2.jpl.nasa.gov/srtm/spie_1998.pdf
12) http://www2.jpl.nasa.gov/srtm/aoda.html
13) Yuhsyen Shen, Scott Shaffer, Rolando L. Jordan, “Shuttle Radar Topography Mission (SRTM) flight system design and operations overview,” Proceedings of SPIE, 'Microwave Remote Sensing of the Atmosphere and Environment II,' Thomas T. Wilheit; Harunobu Masuko; Hiroyuki Wakabayashi; Eds.,Vol. 4152, Oct. 9-12, 2000, Sendai, Japan, pp. 167-178
14) http://www2.jpl.nasa.gov/srtm/instr.htm
15) “Operating the X-band SAR Interferometer of the SRTM,” Proceedings of the IGARSS 2000 (International Geoscience and Remote Sensing Symposium), Honolulu, Hawaii, July 24-28, 2000
16) Marian Werner, “Shuttle radar topography mission (SRTM): experience with the X-band SAR interferometer,” Proceedings of the International Conference on Radar, 2001, Beijing, China, Oct. 15-18, 2001, Volume, Issue, 2001, pp. 634-638
17) Marian Werner, “Shuttle Radar Topography Mission (SRTM)-Mission Overview,” Proceedings of EUSAR 2000, 3rd European Conference on Synthetic Aperture Radar, Mai 23-25, 2000, Munich, Germany, pp.209 - 212
18) M. U. Werner, J. Heinstadt, “A Spaceborne X-band Single Pass Interferometric SAR Antenna System,” ESTEC Workshop on Large Antennas for Radio Astronomy, Noordwijk, NL, Feb. 28-29, 1996
19) K. B. Klein, M. U. Werner, “System Performance Monitoring for X-SAR/SRTM,” EUSAR'98, VDE-Verlag, May 25-27, 1998, Friedrichshafen, Germany, pp. 383-386
20) R. Bamler, “The SRTM Mission: A World-Wide 30 m Resolution DEM for SAR Interferometry for 11 Days,” Photogrammetric Week '99, URL: http://www.ifp.uni-stuttgart.de/publications/phowo99/bamler.pdf
21) http://www.shuttlepresskit.com/STS-99/payload46.htm
22) M. Eineder, R. Bamler, N. Adam, S. Suchandt, H. Breit, U. Steinbrecher, B. Rabus, W. Knöpfle, “Analysis of SRTM Interferometric X-band Data: First Results,” Proceedings of the IGARSS 2000 (International Geoscience and Remote Sensing Symposium), Honolulu, Hawaii, July 24-28, 2000
23) “The SRTM global Mapping Mission... almost 2 years later!,” Jan. 24, 2002, URL: http://spatialnews.geocomm.com/features/srtm_jan2002/
24) http://srtm.det.unifi.it/eng_vers/index1.htm
25) P. .Audenino, L. Rognant, “Validation of SRTM-X Digital Elevation Model toward risk applications,” Proceedings of EUSAR 2004, Ulm, Germany, May 25-27, 2004
26) K. Schulz, E. Cadario, U. Soergel, U. Thoennessen, “Comparison of digital height models with different quality concerning the usage in SAR image analysis,” Proceedings of EUSAR 2004, Ulm, Germany, May 25-27, 2004
27) http://www2.jpl.nasa.gov/srtm/index.html
28) http://www.dlr.de/caf/en/desktopdefault.aspx/tabid-5515/9214_read-17716/
29) http://photojournal.jpl.nasa.gov/mission/SRTM
31) http://www2.jpl.nasa.gov/srtm/dataprod.htm#Gallery
32) http://srtm.usgs.gov/index.php
33) Stephanie Potts. “Project 3: Acquiring Geographic Data from the Shuttle Radar Topography Mission,” Dec. 2006, URL: https://web.archive.org/web/20110324232923/http://www.personal.psu.edu/sap258/GEOG482/Project3/SRTM_Project3.html
34) ”Fossil Fjords in Namibia,” NASA Earth Observatory, Image of the Day for 14 September 2021, URL: https://earthobservatory.nasa.gov/images/148822/fossil-fjords-in-namibia
35) “Elevation models from SRTM now available for download free of charge,” DLR, May 25, 2011, URL: http://www.dlr.de/dlr/en/desktopdefault.aspx/tabid-10080/150_read-817/
36) Wolfgang Keydel, David Hounam, Regina, Pac, Marian Werner, “X-SAR/SRTM - Part of a Global Earth Mapping Mission,” Annex to 'Basics of Normal and Differential SAR Interferometry,' EUSAR Tutorial 2004, Proceedings of EUSAR 2004, Ulm, Germany, May 25-27, 2004, URL: http://ftp.rta.nato.int/public//PubFullText/RTO/MP/RTO-MP-061///MP-061-32.pdf
37) Marian Werner, Michael Eineder, “The X-SAR/SRTM Project Calibration Phase,” Proceedings of X-SAR Science Workshop, Florence, Italy, Sept. 17, 2001
38) K. Sarabandi, C. G. Brown, L. Pierce, D. Zahn, “Calibration of the Shuttle Radar Topography Mission Using Point and Distributed Targets,” Proceedings of the IGARSS 2000 (International Geoscience and Remote Sensing Symposium), Honolulu, Hawaii, July 24-28, 2000
39) K. Sarabandi, C. G. Brown, L. Pierce, D. Zahn, R. Azadegan, K. Buell, M. Casciato, I. Koh, D. Lawrence, M. Park, “Calibration and Validation of the Shuttle Radar Topography Mission Height Data for Southeastern Michigan,” Proceedings of IGARSS 2002 and the 24th Canadian Symposium on Remote Sensing, Toronto, Canada, June 24-28, 2002
40) Y. Lou, Y. Kim, J. van Zyl, L. Maldonado, T. Miller, E. O’Leary, G. Romero, W. Skotnicki, V. Taylor, “The NASA/JPL Airborne Synthetic Aperture Radar’s 1996 PacRim Deployment,” Geoscience and Remote Sensing, Vol. 3, Aug. 3-7,1997, pp.1404-1406
The information compiled and edited in this article was provided by Herbert J. Kramer from his documentation of: ”Observation of the Earth and Its Environment: Survey of Missions and Sensors” (Springer Verlag) as well as many other sources after the publication of the 4th edition in 2002. - Comments and corrections to this article are always welcome for further updates (eoportal@symbios.space).
Mission overview Launch Sensor Complement Ground operations SRTM observation coverage Ground Segment References Back to top