Solar Orbiter Mission
Non-EO
ESA
NASA
Quick facts
Overview
Mission type | Non-EO |
Agency | ESA, NASA |
Solar Orbiter MissionSpacecraft Launch Mission Status Sensor Complement References
Solar Orbiter is a satellite mission of ESA (in the footsteps of Helios, Ulysses, SOHO and the Cluster missions) to explore the inner regions of the sun and the heliosphere from a near-sun orbit. Solar Orbiter is part of the ESA's Science Program Cosmic Vision 2015-2025. The Solar Orbiter project was initially selected by ESA's Science Program Committee in Oct. 2000 and re-confirmed as part of the ESA program in 2003. The Solar Orbiter mission of ESA and the SPP (Solar Probe Plus) mission of NASA (launch scheduled for 2018) are part of the common GHO (Great Heliophysics Observatory) program.
In 2011, the Solar Orbiter mission has undergone extensive study over a period of more than 10 years, both internally in ESA and in industry. This has resulted in a mature, detailed design that satisfies the requirements placed on the mission by the science objectives and addresses the key risk areas. - ESA's Science Program Committee selected the Solar Orbiter mission for implementation on October 4, 2011 with a launch scheduled for 2017. 1) 2) 3) 4)
ESA-NASA collaboration: NASA and ESA have a mutual interest in exploring the near-Sun environment to improve the understanding of how the Sun determines the environment of the inner solar system and, more broadly, generates the heliosphere itself, and how fundamental plasma physical processes operate near the Sun. A NASA-ESA MOU (Memorandum of Understanding) for a Solar Orbiter mission cooperation was signed in March 2012. 5)
For Solar Orbiter, also referred to as SolO in the literature, ESA is providing the spacecraft bus, integration of the instruments onto the bus, mission operations, and overall science operations. NASA is providing an EELV (Evolved Expendable Launch Vehicle) that will place the Solar Orbiter spacecraft into an inner heliospheric orbit with perihelia ranging from 0.28 to 0.38 AU and aphelia from 0.73 to 0.92 AU. The SolO nominal science mission will begin with a series of perihelion passes where the spacecraft is nearly co-rotating with the Sun. It will then use multiple Venus gravity assist maneuvers to move its orbital inclination to progressively higher helio latitudes, reaching 25° by the end of the nominal prime mission phase and around 34° by the end of the extended mission.
The overall objective is to provide close-up views of the sun's high latitude regions - to study fundamental physical processes common to solar, astrophysical and laboratory plasmas. The Solar Orbiter will, through a novel orbital design and its state-of-the-art instruments, provide exactly the observations required. 6) 7) 8) 9) 10) 11) 12) 13) 14)
• During the nominal operational lifetime, the Solar Orbiter operational orbit shall have the following parameters:
- Minimum perihelion radius larger than 0.28 AU to maximize the reuse of BepiColombo technology
- Perihelion radius within 0.30 AU in order to guarantee multiple observations close to the Sun
- Inclination with respect to solar equator increasing to a minimum of 25º (with a goal of 35º in the extended operational phase).
• At minimum perihelion passage, the spacecraft shall maintain a relative angular motion with respect to the solar surface such that individual solar surface features can be tracked for periods approaching one solar rotation.
• The Solar Orbiter system lifetime shall be compatible with a launch delay of 19 months (launch window locked to the next Venus gravitational assist opportunity).
Scientific requirements: The overarching objective of the Solar Orbiter mission is to address the central question of heliophysics: How does the Sun create and control the heliosphere? Achieving this objective is the next critical step in an overall strategy to address one of the fundamental questions in the Cosmic Vision theme: How does the Solar System work? To this end, the Solar Orbiter will use a carefully selected combination of in-situ and remote-sensing instrumentation, a unique orbit and mission design, and a well-planned observational strategy to explore systematically the region where the solar wind is born and heliospheric structures are formed.
The broad question that defines the overarching objective of the Solar Orbiter mission is broken down into four interrelated scientific questions:
1) How and where do the solar wind plasma and magnetic field originate in the corona?
2) How do transients drive heliospheric variability?
3) How do solar eruptions produce energetic particle radiation that fills the heliosphere?
4) How does the solar dynamo work and drive connections between the Sun and heliosphere?
Common to all of these questions is the requirement that Solar Orbiter make in-situ measurements of the solar wind plasma, fields, waves, and energetic particles close enough to the Sun that they are still relatively pristine and have not had their properties modified by dynamical evolution during their propagation. The Solar Orbiter must also relate these in-situ measurements back to their source regions and structures on the Sun through simultaneous, high-resolution imaging and spectroscopic observations both in and out of the ecliptic plane.
Basic Mission Requirements of Solar Orbiter
- Total cruise phase duration < 3 years (goal) with valuable science during the cruise phase
- Orbital period in 3:2 resonance with Venus
- At least one orbit with perihelion radius < 0.25 AU and > 0.20 AU (science phase)
- Inclination with respect to solar equator increasing to a minimum of 30º
- During the extended operational lifetime, the Solar Orbiter operational orbit shall reach an inclination with respect to solar equator not lower than 35º (goal)
- Support a payload of 180 kg and 180 W (including 20% maturity margins) with a data rate of 100 kbit/s
- Provide onboard mass memory and communications with a single ESA deep-space ground station (New Norcia, Western Australia) in support of the science observations
- Fail-safe onboard autonomous operations during the perihelion passages (15 days without ground contact, in extremely harsh thermal environment).
The mission includes a nominal mission phase and a potential extended mission phase (corresponding to 6 solar orbits). The spacecraft consumables and radiation sensitive units shall be sized to meet the duration with extended phase: 9.5 years.
Historical overview of the Solar Orbiter program (Ref. 12):
The Solar Orbiter mission has its origins in a proposal called "Messenger" that was submitted by Richter et al. in 1982 in response to an ESA call for mission ideas. At the meeting "Crossroads for European Solar and Heliospheric Physics" held on Tenerife in March 1998, the heliophysics community recommended to "Launch an ESA Solar Orbiter as ESA's [next flexible] mission, with possible international participation, [for launch] around 2007." The kick-off meeting for a pre-assessment study of the "ESA Solar Orbiter" concept was held at ESTEC on 25 March 1999.
Solar Orbiter was subsequently proposed in 2000 by E. Marsch et al., and was selected by the SPC (Science Program Committee) in October 2000 as a Flexi-mission for launch after BepiColombo (in the 2008-2013 timeframe). A number of internal and industrial studies were then carried out, including parallel system-level Assessment Studies performed in industry between April and December 2004. At its 107th meeting in June 2004, the SPC confirmed the place of Solar Orbiter in the Cosmic Vision program, with the goal of a launch in October 2013 and no later than May 2015.
Work continued on the mission and payload definition throughout 2005 and 2006, and at its meeting in February 2007, SPC asked the Executive to find ways to implement Solar Orbiter within a financial envelope of 300 M€ (at 2006 EC), while keeping a realistic contingency margin. In response to this request, a Joint Science and Technology Definition Team (JSTDT) comprising scientists and engineers appointed by ESA and NASA, studied the benefits to be gained by combining ESA's Solar Orbiter mission and NASA's Solar Sentinels into a joint program. This led to the release of an ESA Announcement of Opportunity for the Solar Orbiter Payload on on Sept. 18 2007 and a NASA SMEX/FOSO (Small Explorer/Focused Opportunity for Solar Orbiter) AO that was issued on 22 October 2007.
A major change in the progress of Solar Orbiter occurred in November 2008, when the SPC decided to integrate the Solar Orbiter into the first planning cycle of Cosmic Vision 2015-25 as an M-mission candidate for the first launch opportunity in 2017. In addition, in view of NASA's high prioritization of SPP (Solar Probe Plus) in its Living With a Star program (compared with Sentinels), and the strong science synergies between Solar Orbiter and SPP, ESA called for an independent review of the PRC's (Payload Review Committee) recommended payload, now in the context of a joint Solar Orbiter-SPP scientific program. The joint ESA-NASA review panel confirmed the validity of the recommended payload in its report of March 2009. As a result, the instrument selections recommended by the PRC in 2008 were formally announced on 20 March 2009. In parallel, NASA announced the results of the FOSO selection, and selected 2 instruments and portions of 2 instruments to be included in the Solar Orbiter payload.
At its 128th meeting on Feb. 17-18, 2010, ESA's Science Program Committee decided that the Solar Orbiter be one of the three M-class candidates to proceed into definition phase and made a further programmatic change by endorsing a "fast track" approach outlined in ESA/SPC(2010)3, rev. 1. This approach was based on the scientific acceptability of increasing the minimum perihelion to ~0.28 AU and making maximum reuse of Bepi-Colombo technologies. Furthermore, it called for the start of the spacecraft implementation program (Phase B2) in early 2011, with a view, if selected, to mission approval and adoption by the SPC in October 2011, leading to a launch in 2017. In line with this approach, the industrial Phase B2 activities were kicked-off in February 2011.
In March 2011, NASA informed ESA that it had decided to reduce its contribution to the payload to 1 full instrument and 1 sensor. Specifically, the Spectral Imaging of the Coronal Environment and Suprathermal Ion Spectrograph investigations would not be funded. Given the scientific importance of these investigations, the SPC decided that, should Solar Orbiter be selected, the SPICE and SIS measurement capabilities should be recovered through the inclusion of European-led instruments in the payload, procured under ESA's responsibility.
Solar Orbiter was ultimately selected and adopted as the first M-class mission of ESA's Cosmic Vision program by the SPC on October 4, 2011.
Spacecraft
As with all spacecraft, mass and volume are at a premium due to launch vehicle constraints; however, the Solar Orbiter main spacecraft body is further constrained due to the fact that a sizable portion of the budget is taken up by the heat-shield, along with the fact that the spacecraft must be optimized to fit behind the heat-shield with sufficient margin to cover off-pointing cases, e.g. due to spacecraft anomalies. The Solar Orbiter spacecraft main body is approximately 2 m3 (with stowed appendages). With 33 instrument units to accommodate on-board, the allowable volume of each instrument unit must be tightly controlled.
The Solar Orbiter spacecraft configuration is dominated by the presence of the heat shield located at the top of the spacecraft in order to protect the spacecraft from the intense direct solar flux when approaching perihelion. The heat shield is over-sized to provide the required protection to the spacecraft box and externally-mounted units, in combination with the attitude-enforcement function of the FDIR (Failure Detection, Isolation and Recovery). The mechanical platform revolves around a robust, reliable and conventional concept with a central cylinder, four shear walls and six external panels. This concept is inspired by Astrium's Eurostar 3000 spacecraft platform. The design meets the Solar Orbiter's mission requirements according to a low-risk and low-cost philosophy.
In April 2012, ESA awarded a contract to build its next-generation Sun explorer to Airbus DS (former Astrium UK, Stevenage). Astrium UK will lead a team of European companies who will supply various parts of the spacecraft. 15) 16) 17) 18)
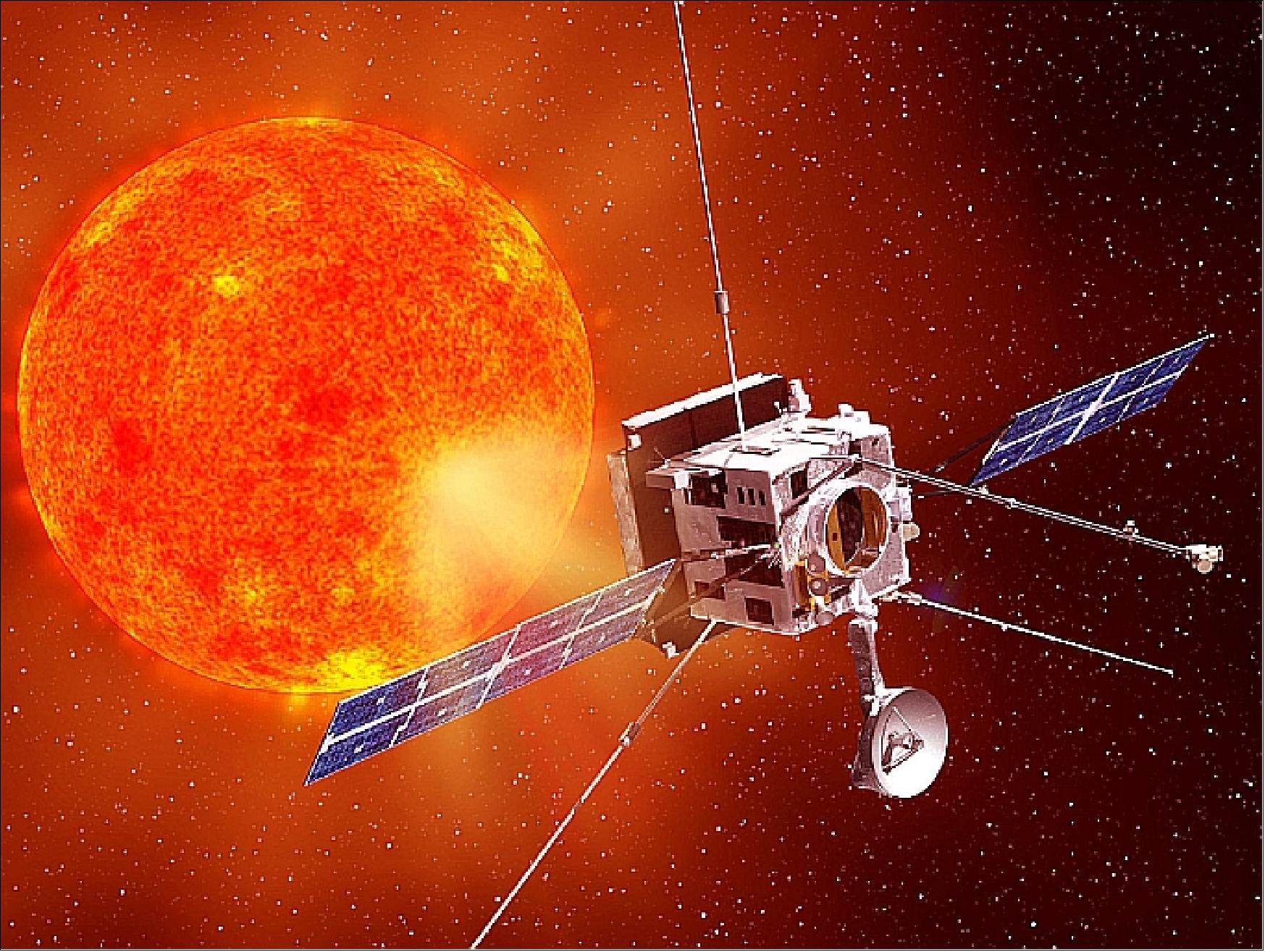
The spacecraft and mission PDR (Preliminary Design Review) was completed on March 7, 2012.Following contract negotiations with the prime contractor, Phase-B2/C/D is proceeding with subsystem-level procurement and several lower-tier procurements.
The spacecraft is three-axis stabilized and always sun-pointed. Given the extreme thermal conditions at 45 solar radii (or 0.22 AU), equivalent to about 20 solar constants or approximately 28 kW/m2, a phenomenal amount of power from which the majority of the spacecraft must be protected. The thermal design of the spacecraft has been considered in detail. Accordingly, the bulk of the spacecraft is protected from the sun by a local heatshield (also referred to as sunshield) on the +X panel face of the spacecraft, combined with a stringent maintenance of a sun-pointing attitude for the spacecraft at all times during periods close to the sun (below ~0.7 AU). 19) 20) 21)
The spacecraft configuration is based on a square structure housing a simple mono-propellant propulsion system with no main engine . Due to the stringent environment encountered on the heliocentric orbits, the spacecraft is always sun-pointed and protected from solar irradiation by a heatshield. This heatshield covers the spacecraft bus and some of the external components such as in-situ instruments. It contains aperture openings providing the required field of view (FOV) for the remote sensing instruments. 22)
The avionics architecture is based on segregated processing functions of the platform and the payload data. The OBMU (On-Board Management Unit) is in charge of the spacecraft command / control, running the DHS (Data Handling Subsystem), AOCS and mission software component, and housing the interfaces with the platform equipments and the payload support unit. The PDPU (Payload Data Processing Unit) supports all functions of the sensor complement. Onboard communications is based on one MIL-STD-1553B bus for the data communications between the OBMU and the platform units, and on a SpaceWire network. 23)
SpaceWire has been selected as the sole communication interface between each of the instruments and the spacecraft DHS (Data Handling Subsystem). It also provides a key interface within the DHS itself, between are the OBC (On-Board Computer ) and the SSMM (Solid State Mass Memory).
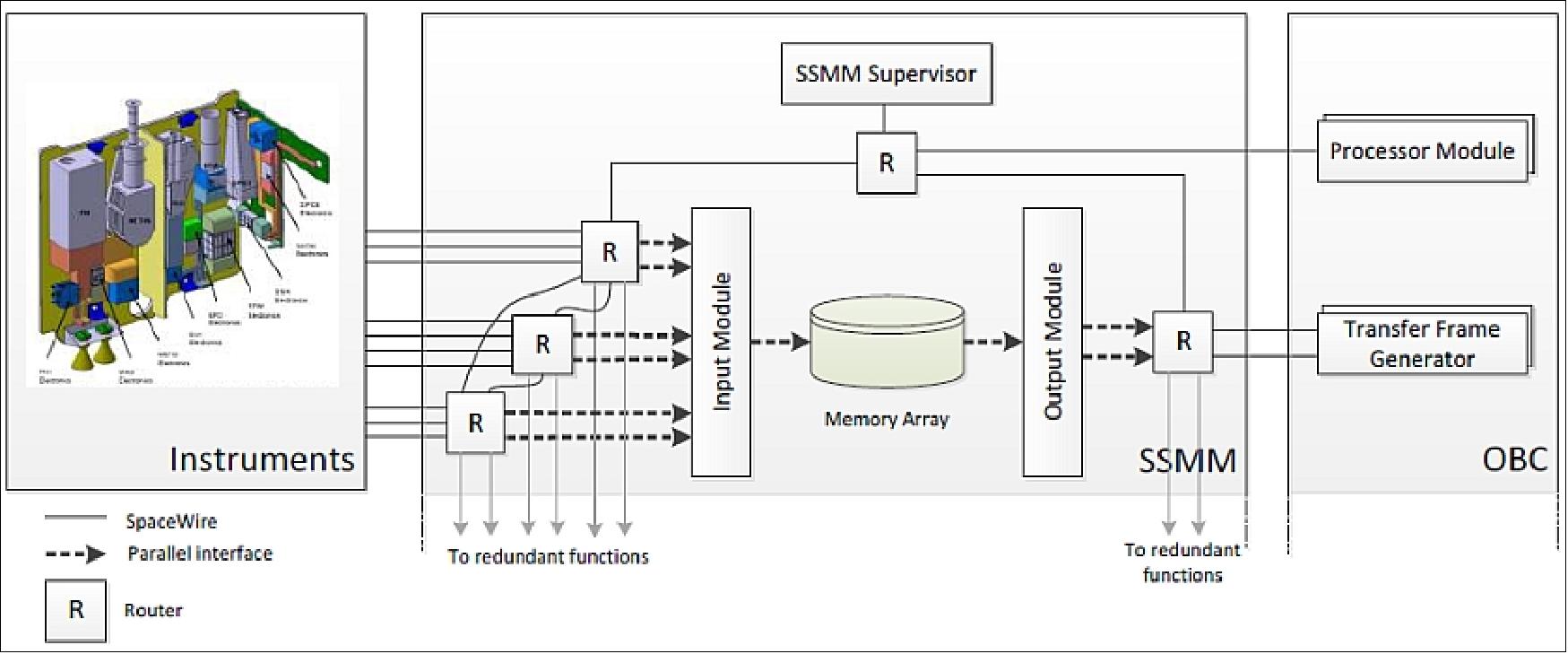
AOCS (Attitude and Orbit Control Subsystem): The AOCS employs an autonomous star tracker, gyros, and sun sensors for attitude acquisition and safe mode sensing; actuation is provided by reaction wheels and thrusters. The AOCS baseline architecture also includes a hard-wired safe mode using a sun sensor and a coarse gyro aimed at recovering as fast as possible the sun-pointed attitude in case of contingency, which is essential for the spacecraft thermal safety.
The AOCS constitutes a suite of components that in close interaction with the rest of the spacecraft controls the orientation and stability of the spacecraft, and executes the ground requested velocity changes for adjustment of the otherwise ballistic trajectory. This function includes the monitoring of its own health, as well as the provision of a reference on selected data related to trajectory and orientation, in order to support control of mechanisms.
A set of primary requirements to the AOCS are:
- Maximum 6.5º off-pointing from the Sun, with maximum 50s off-pointing over 2.3º
- Capacity of fine pointing without star tracker measurements for at least 24 hours
- A fine pointing Absolute Pointing Error of 42 arcsec, with an Attitude Knowledge Error of 25 arcsec. The Pointing Drift Error is specified at 13 arcsec over 24 hours, using 10s integration windows. All figures are applicable to Line of Sight to the Sun, 95% confidence.
The AOCS consists of most of the classical elements found on interplanetary missions, but with the special feature that the onboard computer handles all tasks, such as data handling, thermal control, AOCS and FDIR (Failure Detection, Isolation and Recovery), on a single processing module. The equipment used are two pairs of Fine Sun Sensors, two Inertial Measurement Units, two Star Trackers, four Reaction Wheels, and a redundant bi-propellant propulsion system consisting of 9 thrusters per branch. The Inertial Measurement Units consist of one nominal branch featuring high performance rate measurements from four tetrahedron oriented gyroscopes and a contingency branch providing reduced rate measurement performance. The nominal branch also includes four tetrahedron oriented accelerometer channels. All units are communicated with via two MIL-1553B redundant busses. The units are synchronized to the onboard time reference at a minimum of 8Hz data acquisition, corresponding to the attitude control frequency. 24)
EPS (Electric Power Subsystem): The solar panel design relies on a carbon/carbon substrate with triple junction GaAs cells. The operational temperature of this new solar array technology is expected to be 230ºC. On SolO this can be achieved by implementing a large enough OSR (Optical Surface Reflector) ratio and solar array tilt angle such that the sun incidence angle is high enough to limit the incident solar flux. The EPS architecture employs a regulated power bus. One Li-ion battery is foreseen to cover mission needs during LEOP and Venus gravity assists. - The solar arrays can be rotated about their longitudinal axis to avoid overheating when close to the Sun.
The S/C dimensions are: 2.5 m x 3.0 m x 2.5 m. The pointing stability is better than 3 arcsec/15 min. The total spacecraft wet mass is about 1800 kg, the maximum power demand is ~ 1100 W. The payload suite mass budget is ~190 kg with a payload power consumption of 180 -250 W (depending on the mission phase).
Spacecraft | 3-axis stabilized platform, heat shield, two adjustable solar arrays, dimensions: 2.5 m x 3.0 m x 2.5 m(launch configuration) |
Spacecraft orientation | Sun pointing |
Telemetry band | Dual X-band |
Data downlink | 150 kbit/s (at 1 AU spacecraft-Earth distance) |
Closest perihelion | 0.28 AU (Astronomical Unit), corresponding to a Sun-spacecraft distance of ~ 42 million km. |
Max. heliographic latitude | 25º (nominal mission) / 34–36º (extended mission) |
Launch date | January 2017 (March 2017 and September 2018 back-ups) |
Nominal mission duration | 7 years (including cruise phase) |
Extended mission duration | 3 years |
Post-operations & archiving | 2 years |
Ground station | Malargüe (Argentina), 35 m antenna, 4 to 8 hours/day (effective) |
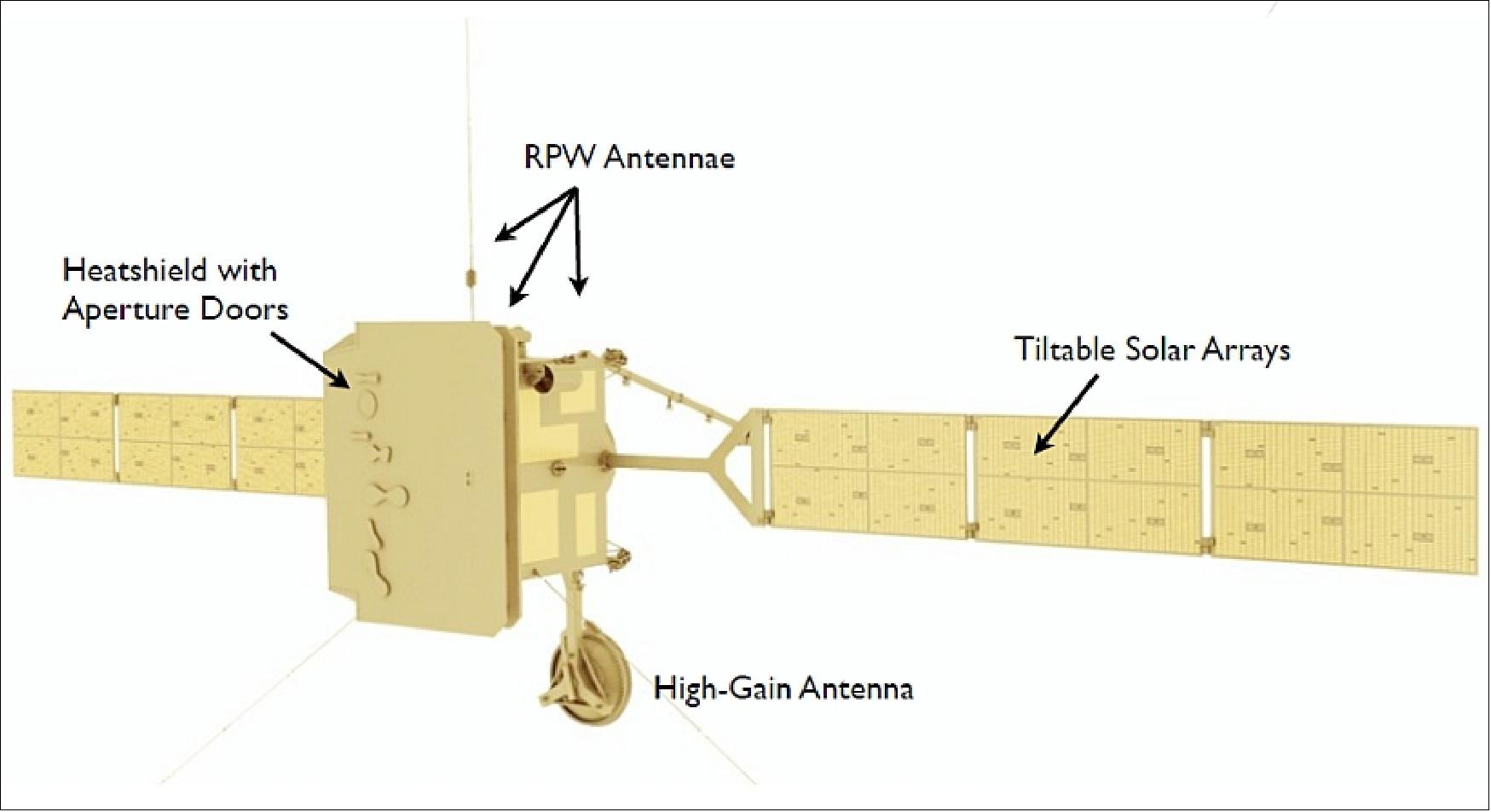
Heatshield Design
The Solar Orbiter thermal control is based on using a sun pointed, flat heat shield to limit the sun flux on the spacecraft structure. By using this approach the elements behind the heat shield will be in a more benign thermal environment. 27)
All external components are shielded from direct solar illumination by the heat shield except for the instruments requiring direct view of the sun and the spacecraft appendages, i.e. the solar arrays, the RPW (Radio & Plasma Wave Analyzer) antennas and the HGA (High Gain Antenna). The heat shield is sized to prevent direct solar illumination on any of the shaded components during nominal pointing and for safe mode events of spacecraft off-pointing up to 6.5º from sun-center. However, the spacecraft must also withstand reflected solar flux and high IR flux from appendages outside of the heat shield shadow cone. In addition, the remote sensing instruments will all receive additional IR flux from the feedthroughs which allow them to view through the heat shield.
The design allocates the heatshield at the top of the spacecraft to free all four lateral walls for high efficiency radiators with good viewing factors towards cold space. A key strategy in the restriction of the Solar Orbiter mission cost is to reuse technology from other programs, primarily of course the BepiColombo program given the environmental similarities. The heatshield requirements call for:
• The heatshield must protect the majority of the spacecraft, including the payload, from the punishing incident solar flux (28 kW/m2 at perihelion)
• At the same time the heatshield must incorporate cut-outs to allow the RS (Remote Sensing)-instrumentation, and the sun sensors, access to the sun.
The definition of ‘protection' is that the heatshield will:
• Limit the overall radiative heat flux to the spacecraft to no more than 30 W in total
• Limit the overall conductive heat flux at all attachment points to the spacecraft to no more than 15 W in total.
The technological challenges of the heatshield were addressed through parallel contracts awarded to TAS-I and Airbus DS (former EADS Astrium) with the goal of design and production of thermal breadboards to demonstrate the concepts.
The essential function of the heatshield was identical in both cases. Each heatshield presents a planar surface to the sun, and relies on using multiple layers with large gaps in-between to facilitate lateral heat rejection to cold space. However the two resulting breadboard concepts were different in a number of key aspects:
- Materials: The choice of material for the outer layer (sunshade) is obviously critical as this effectively sets the temperature of the outer layer and the subsequent performance of the entire heatshield. The TAS-I design employed Carbon-Carbon fabric with an additional Nickel light blocking layer; the Astrium design used Keplacoat© on a Titanium foil.
In the meantime, the initial choice – carbon-fiber fabric – was ruled out. Instead the sunshade team began looking for the answer outside the space business. They found it in the shape of Irish company Enbio and its CoBlast technique, originally developed to coat titanium medical implants. The process works for reactive metals like titanium, aluminum and stainless steel, which possess a surface oxide layer. The team sprays the metal surface with abrasive material to grit-blast this layer off; also included is a second ‘dopant' material possessing whatever characteristics are needed. This simultaneously takes the place of the oxide layer being stripped out. The big advantage is that the new layer ends up bonded, rather than only painted or stuck on. It effectively becomes part of the metal.
The material Enbio will apply to the outermost titanium sheet of Solar Orbiter's multi-layered heatshield is called ‘Solar Black' – a type of black calcium phosphate processed from burnt bone charcoal. 28)
- Support Panel: The Astrium concept used a separate Aluminum support panel for the heatshield in addition to the +X spacecraft panel upon which it is mounted, which allows the heatshield to be treated as a separate item to the spacecraft (highly desirable for programmatic reasons); this is in contrast to the TAS-I design in which the support panel of the heatshield is part of the spacecraft primary structure.
- Number of gaps: The Astrium design utilized a single gap between the sunshade and the support panel, with an additional gap between the support panel and the +X panel of the spacecraft. In contrast the TAS-I design employed 3 equidistant space gaps between 4 layers.
- Layer support: The Astrium design relied on pretensioned lashes in order to provide stiffness to the sunshade layer and a high degree of planarity (this improves thermal performance). In contrast the TAS-I design favored loose support of the layers by rigid Star Brackets – although the planarity of the layers is reduced, the mechanical performance of this approach is considerably better.
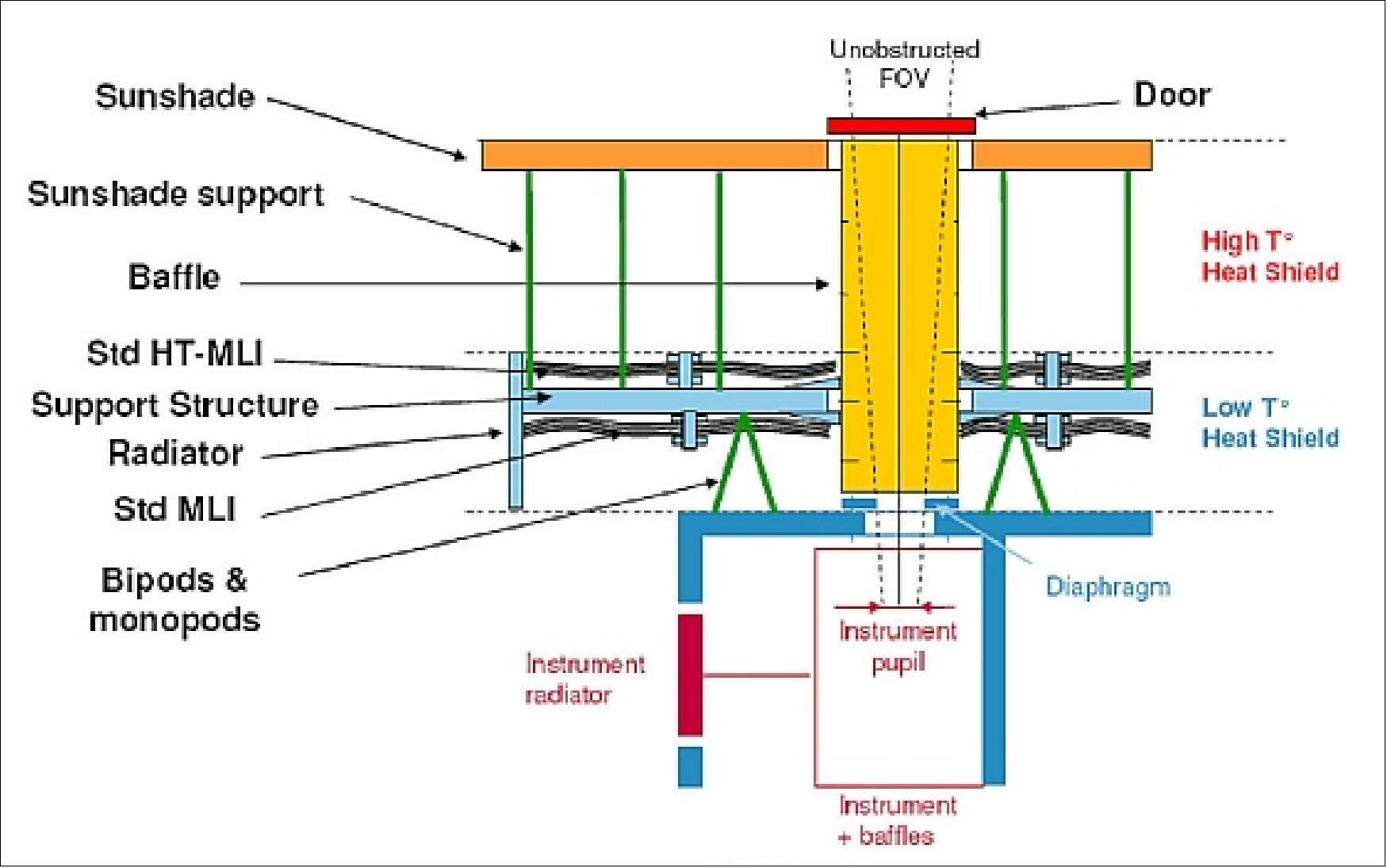
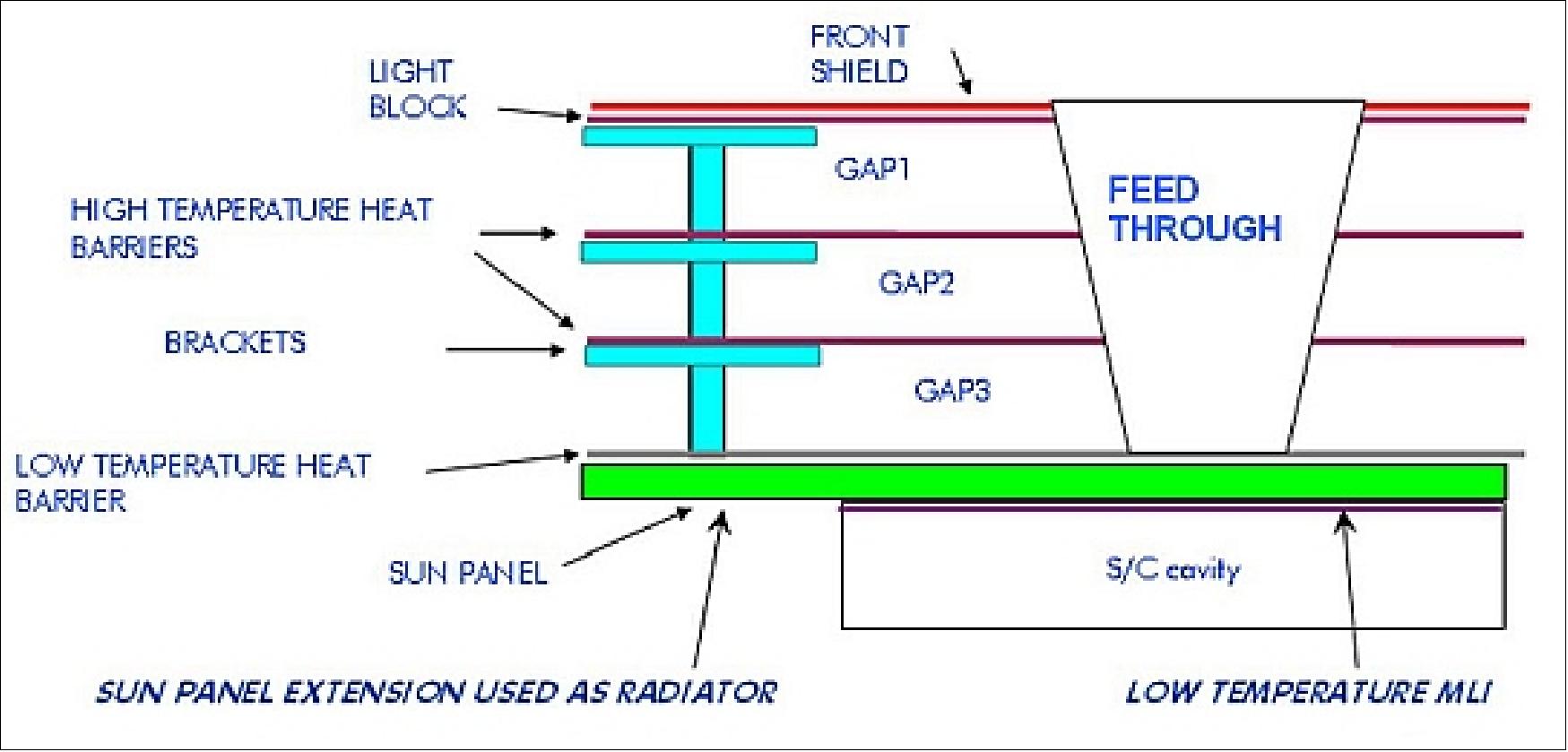
Feedthrough doors and mechanisms: A critical component of the overall heatshield design is the feedthrough and door arrangement that allows the RS-instruments to see through the heatshield. The generic design is applicable for all the RS-instrument feedthroughs: a cylindrical feedthrough with internal vanes to specify the FOV of the instrument. Each feedthrough is mechanically supported by an interface to the support panel of the heatshield, and in turn the feedthroughs provide local support to the sunshade (uppermost) layer of the heatshield through a second interface.
The doors are made of Titanium, with a ‘duck-foot' design incorporating radial spars. The door does not provide any contamination control, it has only a light-blocking function, and consequently does not touch the structure underneath. Instead it is displaced above the feedthrough by ~1 mm, a sizing which ensures non-interaction of the door and feedthrough during launch. The accuracy of the door operation is not critical, as long as the door completely covers the aperture when it is required to do so. A launch lock is present at the door to constrain rotation during launch.
RF communications: The subsystem consists of a redundant set of transponders using X-band for the uplink, and X-band and Ka-band for the downlink. Depending on the mission phases, the transponders can be routed via RF switches to different antennas. The telecommunication subsystem provides hot redundancy for the receiving function and cold redundancy for the transmitting function. One steerable HGA (High Gain Antenna) is being used to support the X-band services for engineering data, and the Ka-band for the science data transmissions.
The X-DST (X-band Deep Space Transponder) is designed and developed by TAS-I (Thales Alenia Space, Italy). The digital platform (whosedesign is inspired by the software-defined radio concept) features a system-on-chip based DSP core, implementing on the same chip all the X-DST signal processing algorithms. 29)
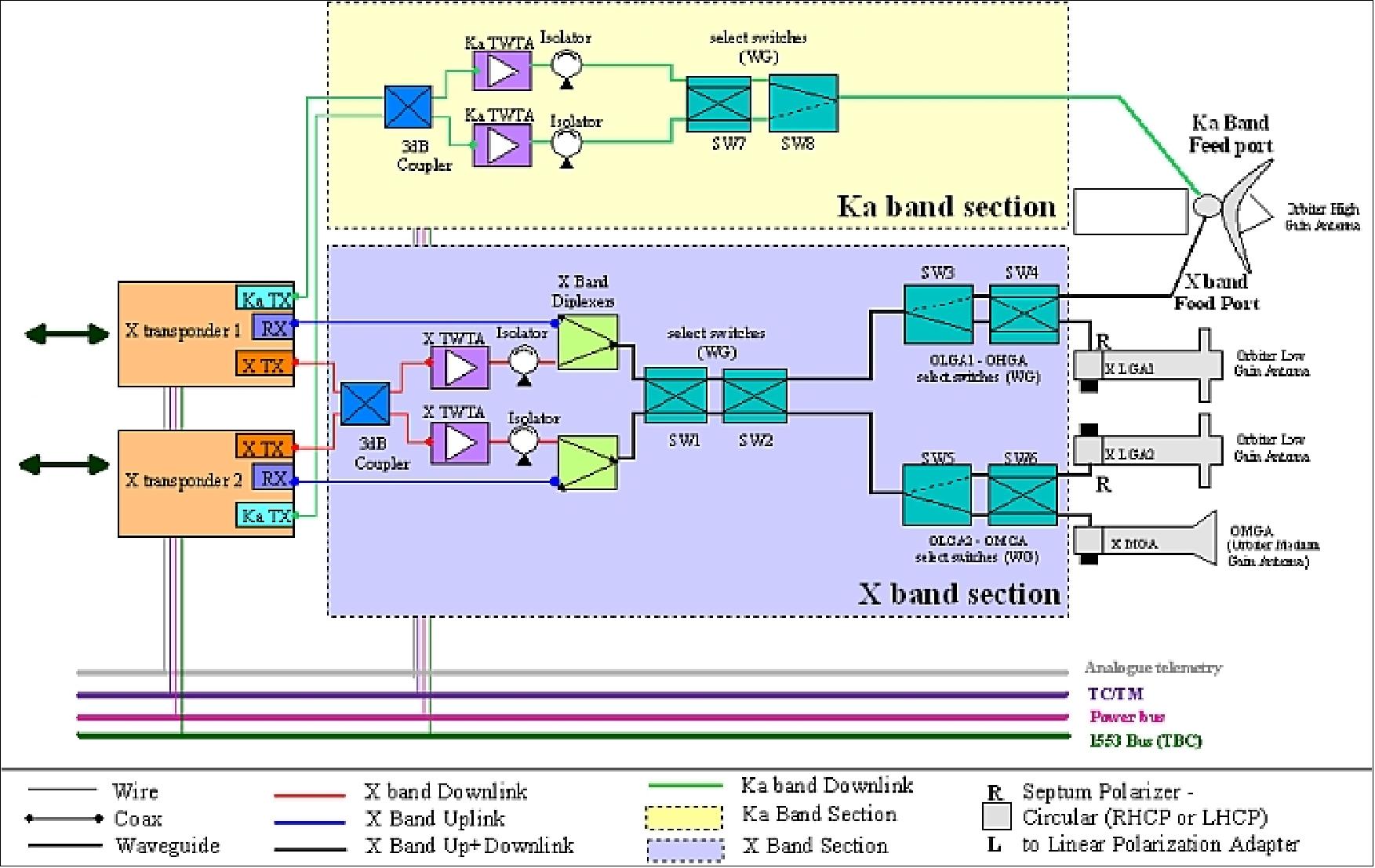
The operations concept is such that the instrument data will be stored in a SSMM (Solid State Mass Memory), for later downlink during daily ground station passes of 8 hours. The science data is downlinked in X-band via the high gain antenna. During the 10 day science windows, the allocation for the nominal average data generation rate of the full payload is 120 kbit/s. This is also controlled via an allocation of the average per instrument. For the remote sensing instruments in particular, their allocation is insufficient to downlink the full raw data and therefore their designs are such as to allow pre-processing, data reduction, selection and associated internal data storage in order to ensure that optimum use is made of the TM bandwidth to downlink the best data. This is not only important for each instrument individually, but for the mission as a whole, as the overriding science objectives rely on combining observations of the same phenomenon from different instruments.
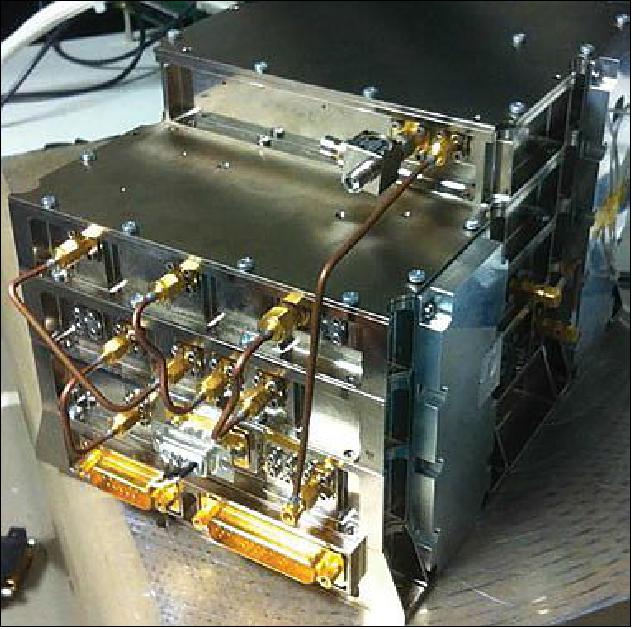
Thermal Architecture of Spacecraft
The TCS (Thermal Control Subsystem) of the spacecraft represents the main design challenge, a critical element for spacecraft integrity and performance for a large proportion of the mission duration. The fundamental Solar Orbiter thermal requirement stipulates that the TCS will support payload and spacecraft subsystems such that it is designed to withstand all thermal environments encountered during the entire life of the mission. The selected approach is to rely on a sun-pointed spacecraft with the spacecraft protected from solar flux by the heatshield, and on specific technologies for the remaining exposed parts, such as the solar panels, communication antennas, and the heatshield. 31)
The heat rejection efficiency of the heatshield permits a quasi-decoupling of the spacecraft body from the direct sun irradiation (flux density of up to 28 kW/m2 at 0.2 AU).The heatshield is made with a highly reflecting/emissive external layer to dissipate the incident flux as much as possible radiatively.
Note: A number of technology developments required by the Solar Orbiter are common to BepiColombo, an ESA mission to the hot planet Mercury with a planned launch in 2013.
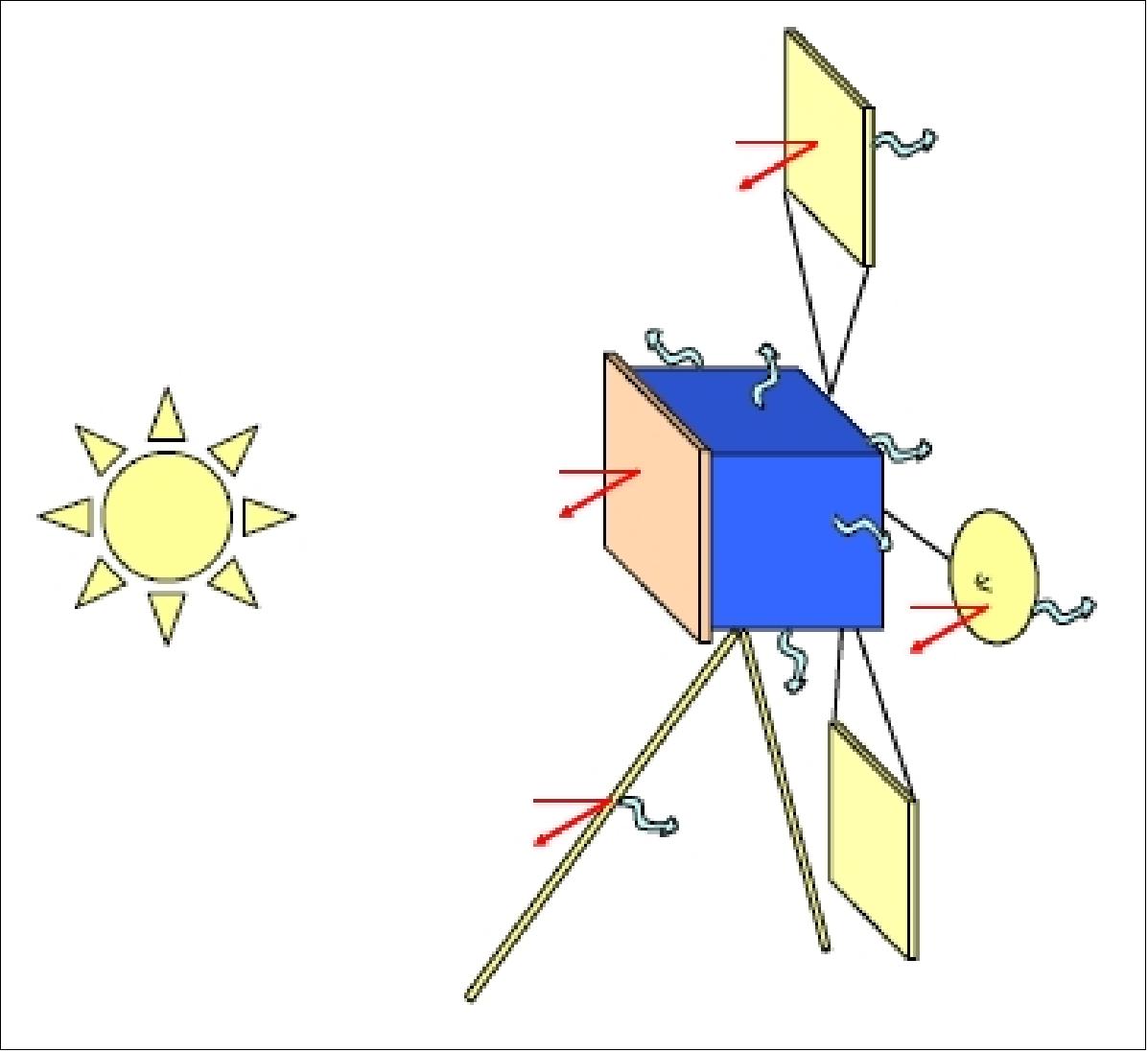
Several payload instrument apertures are implemented through the heatshield to let the remote sensing instruments observe the sun through baffles, and acquire the incident rays on their sensitive detectors. The instruments are either mounted directly on spacecraft lateral walls (in-situ instruments), and use dissipation transferred from the base plate of the unit to the external radiator, or mounted on the spacecraft shear walls (remote-sensing instruments) and use a conductive link from the instruments to the radiators viewing cold space, mounted on external walls, or use dedicated fluid loop pipes. Other radiators accommodated on the lateral walls of the spacecraft are used to cool down internal equipment that dissipate heat or receive solar flux (Figure 9).
The heatshield itself is an innovative and the most sophisticated piece of hardware on SolO. A flat heatshield design is selected and accommodated on top of the spacecraft whose side is always facing to the sun. The heatshield is supported by a structure decoupled from the spacecraft. This structure carries the remote sensing instrument baffles. The baffles cannot be supported by the spacecraft wall since they contain high temperature points or regions. The load-carrying structure is thermally decoupled from the spacecraft wall to minimize conduction loads. The mechanically autonomous heatshield design with respect to the spacecraft is very user-friendly to all AIV (Assembly, Integration and Verification) activities.
The preliminary design of the heatshield outside reflecting layer consists of a white ceramics coating on a titanium (Ti) plate, with an α/ε (absorption/emission) ratio as low as 0.4 - 0.6 at EOL. A multi-layer concept made of polished Ti foils and VDA/VDA (Vapor Deposited Aluminum) kapton foils is proposed for the next layer of insulation to efficiently dissipate the heat and maintain the spacecraft wall at room temperature.
The different layers are held through regularly spaced Ti stand-offs made with limited conductivity towards an Al honeycomb structure to which they are attached. This plate acts as the support structure of the heatshield and is mounted onto the spacecraft wall through a few stand-offs, with a classical MLI (kapton + Dacron) in-between for insulation.
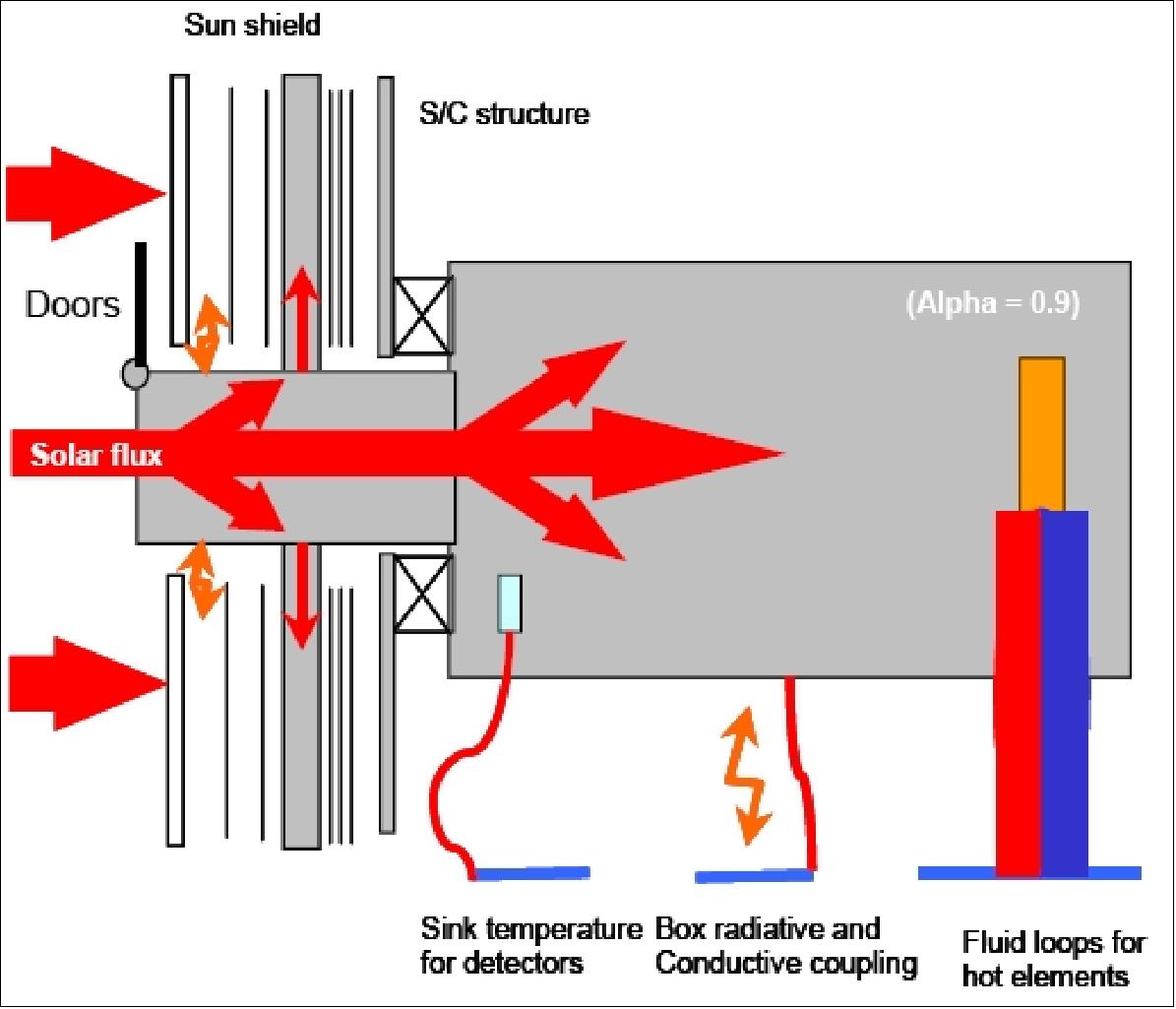
The interfaces between the remote sensing instruments and the heatshield mainly comprise the baffles and instrument shutters aimed at protecting them from contamination and solar flux when they are not operated. The baseline concept is to thermally decouple the baffle from the instrument by attaching it to the heatshield support structure, and to dissipate their heat by conductive coupling through a radiator installed at the edge of the heatshield. Baffles of optical instruments are assumed to be in SiC, while baffles of particle detection instruments (SWA) could be in the same material as the heatshield first layer in order to lower their temperature.
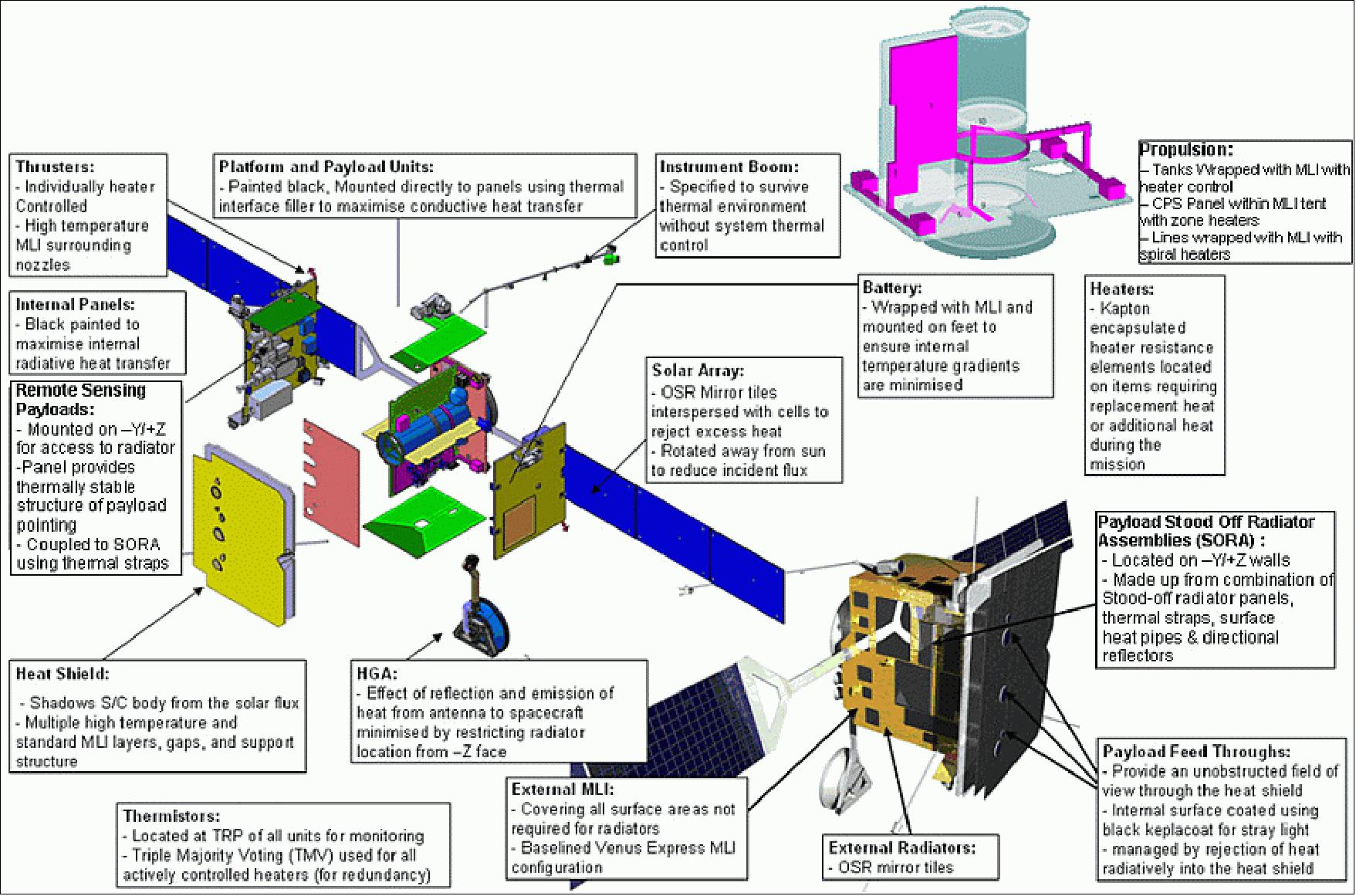
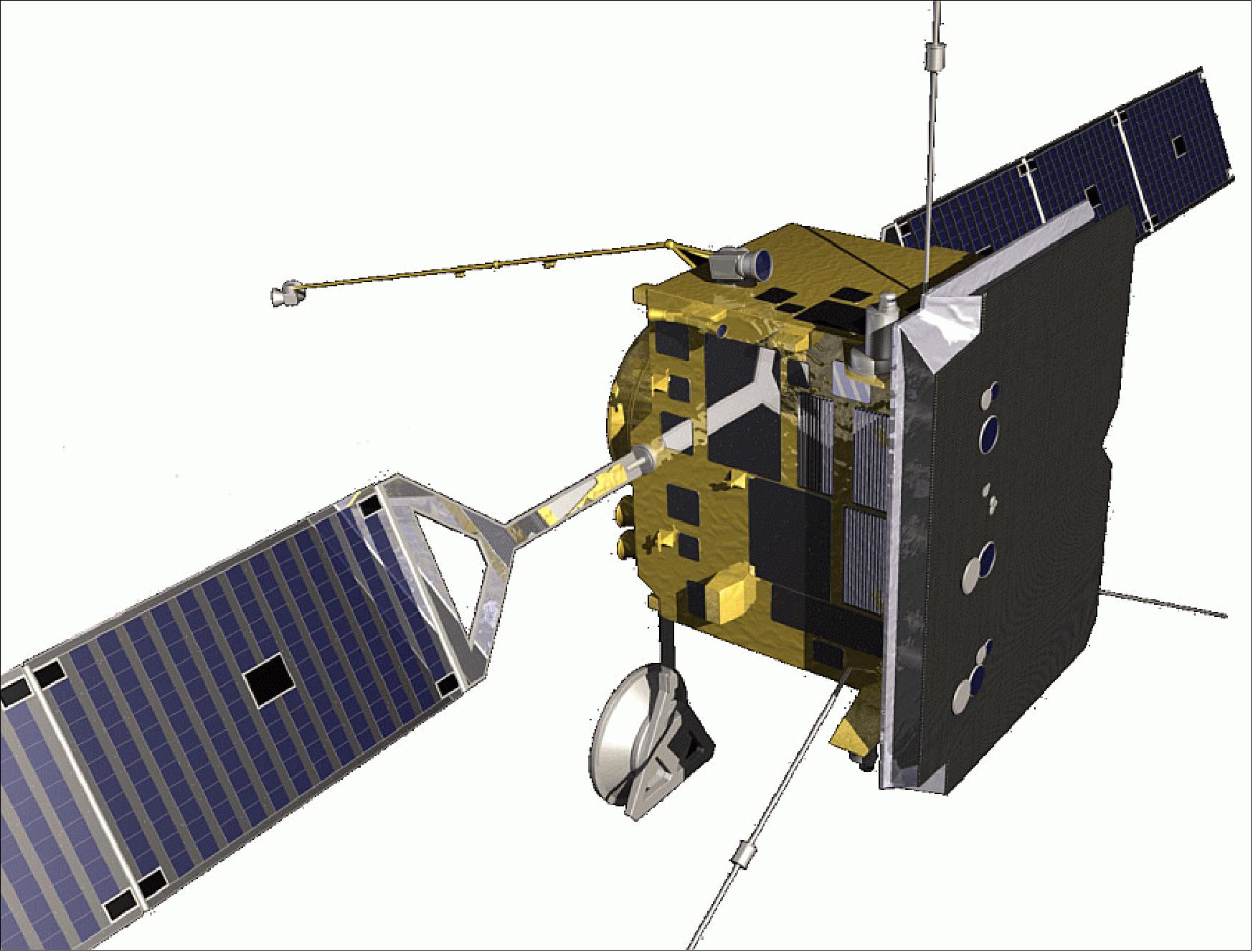
Launch
ESA's Solar Orbiter spacecraft was launched on 10 February 2020 (04:03 GMT) by a NASA-provided Atlas-V 411 vehicle of ULA, designated AV-87, from KSC (Kennedy Space Center) SLC-41 (Space Launch Complex), Cape Canaveral, FL, USA. 32) 33) 34)
- Solar Orbiter, an ESA-led mission with strong NASA participation, will provide the first views of the Sun's uncharted polar regions from high-latitudes, giving unprecedented insight into how our parent star works. This important mission will also investigate the Sun-Earth connection, helping us to better understand and predict periods of stormy space weather.
- Signals from the spacecraft were received at New Norcia ground station in Australia at 06:00 CET (Central European Time), following separation from the launcher upper stage in low Earth orbit.
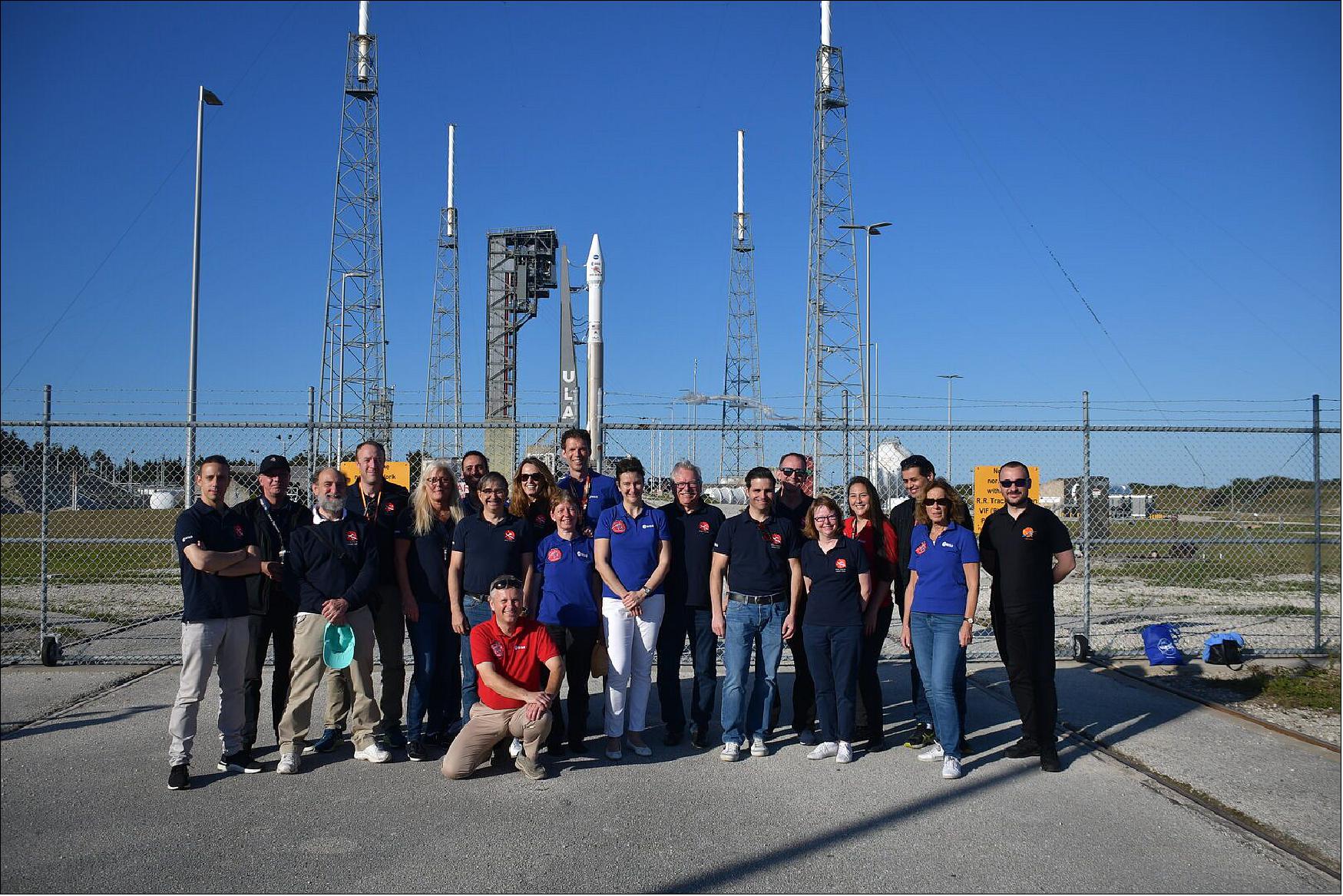
Orbit: The Solar Orbiter will use Venus gravity assists to obtain the high inclinations reaching 35º with respect to the sun's equator (inclined ecliptic orbit) at the end of the cruise phase mission (the cruise phase will last about 3.4 years).
Using SEPM (Solar Electric Propulsion Module) in conjunction with multiple planetary swing-by maneuvers, it will take the Solar Orbiter only two years to reach a perihelion of 45 solar radii with an orbital period of 149 days. Within the nominal 5 year mission phase, the Solar Orbiter will perform several swing-by maneuvers at Venus, in order to increase the inclination of the orbital plane to 30º with respect to the solar equator. During an extended mission phase of about two years, the inclination will be further increased to 38º.
Operating orbit:
- Elliptical orbit around the Sun with a perihelion as low as 0.28 AU and with increasing inclination up to more than 30º with respect to the solar equator.
- Aphelion between 0.8 AU and 0.9 AU
- Co-rotation pass: duration 10 days, with a maximum drift of 50º
- Period about 150 days
- Inclination evolving from 0º-30º (with respect to solar equator), 34º in the extended mission.
During the initial cruise phase, which lasts until November 2021, Solar Orbiter will perform two gravity-assist maneuvers around Venus and one around Earth to alter the spacecraft's trajectory, guiding it towards the innermost regions of the Solar System. At the same time, Solar Orbiter will acquire in situ data and characterize and calibrate its remote-sensing instruments. The first close solar pass will take place in 2022 at around a third of Earth's distance from the Sun. 35)
The spacecraft's orbit has been chosen to be ‘in resonance' with Venus, which means that it will return to the planet's vicinity every few orbits and can again use the planet's gravity to alter or tilt its orbit. Initially Solar Orbiter will be confined to the same plane as the planets, but each encounter of Venus will increase its orbital inclination. For example, after the 2025 Venus encounter it will make its first solar pass at 17º inclination, increasing to 33º during a proposed mission extension phase, bringing even more of the polar regions into direct view.
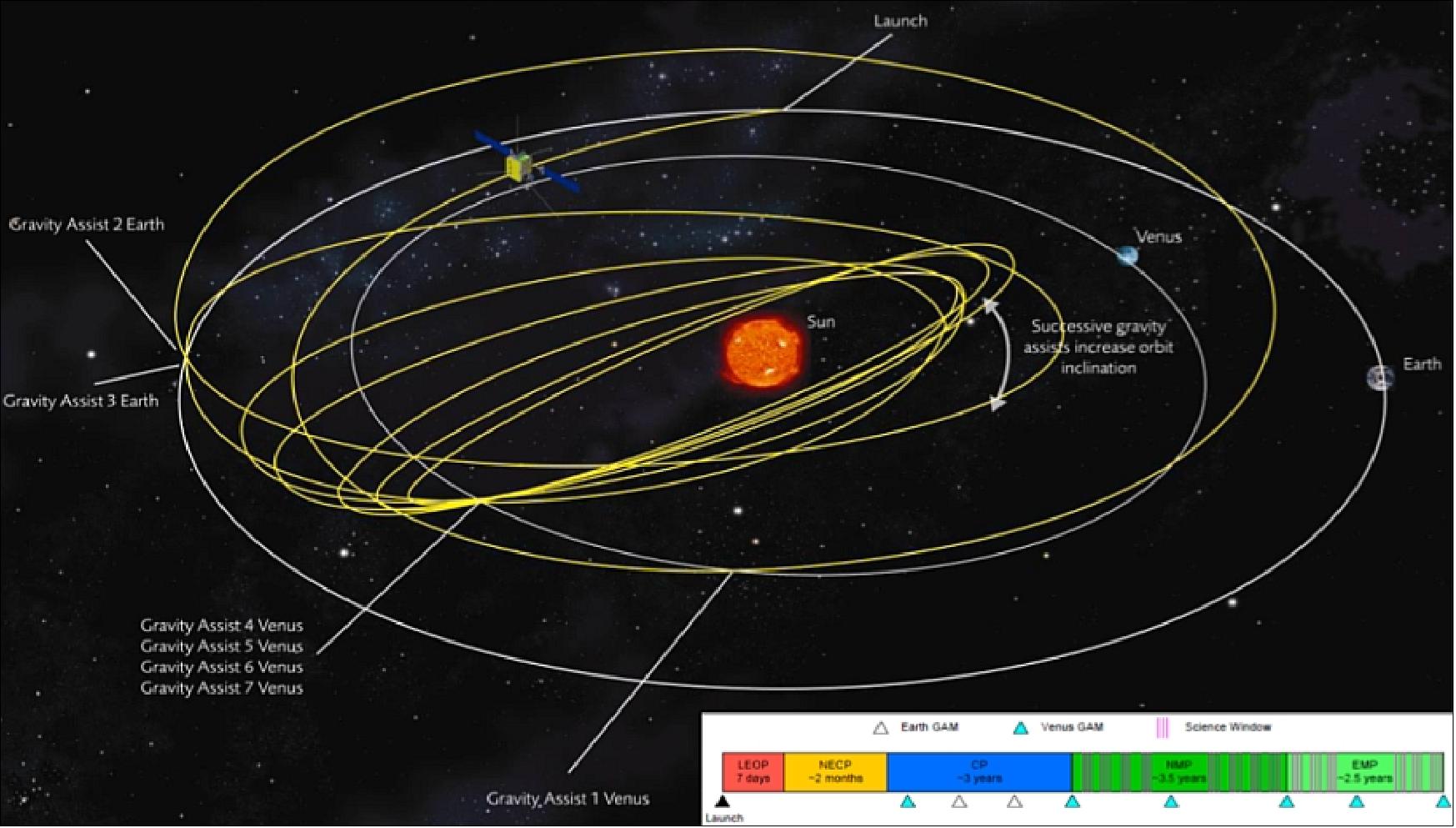
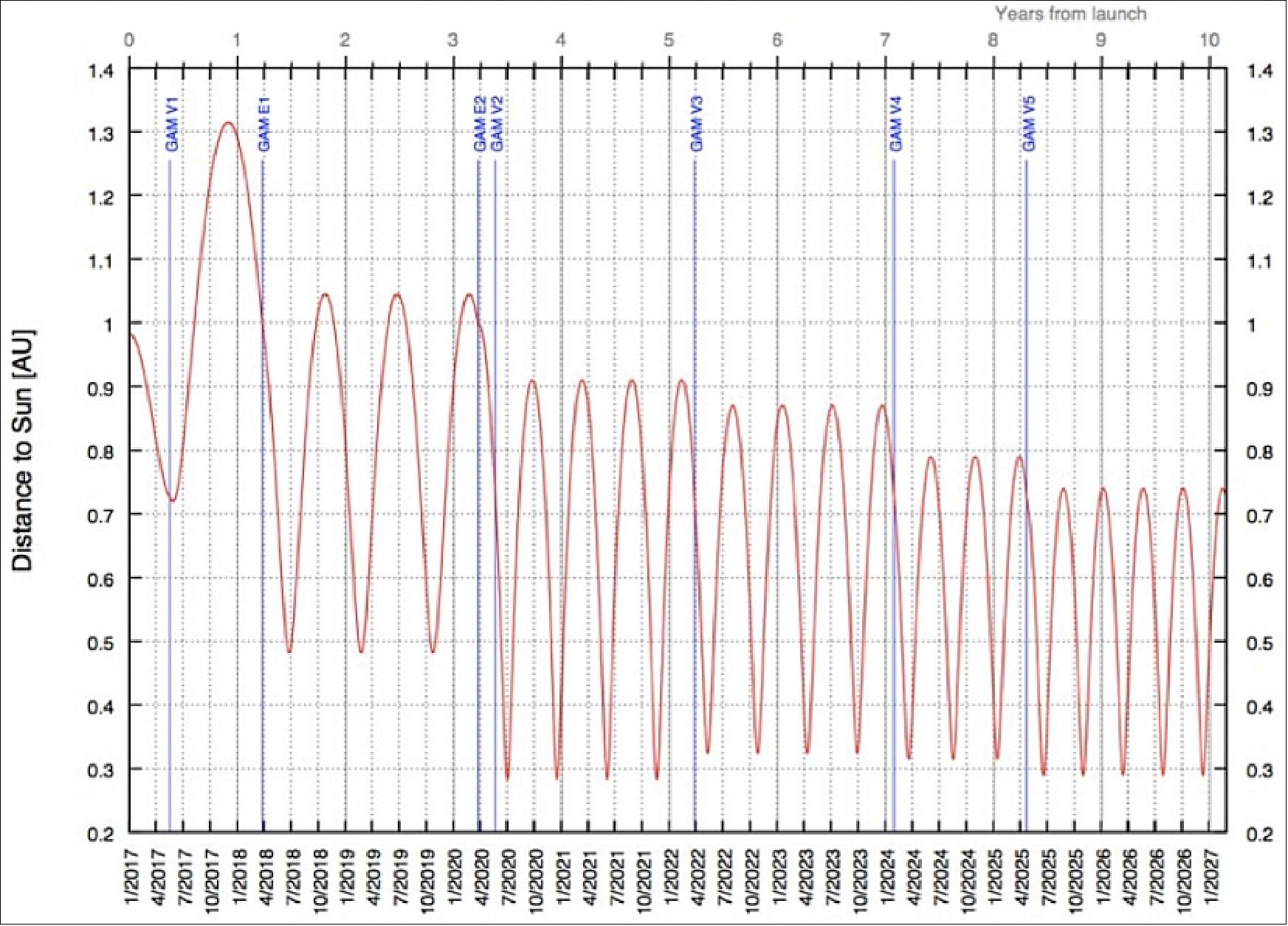
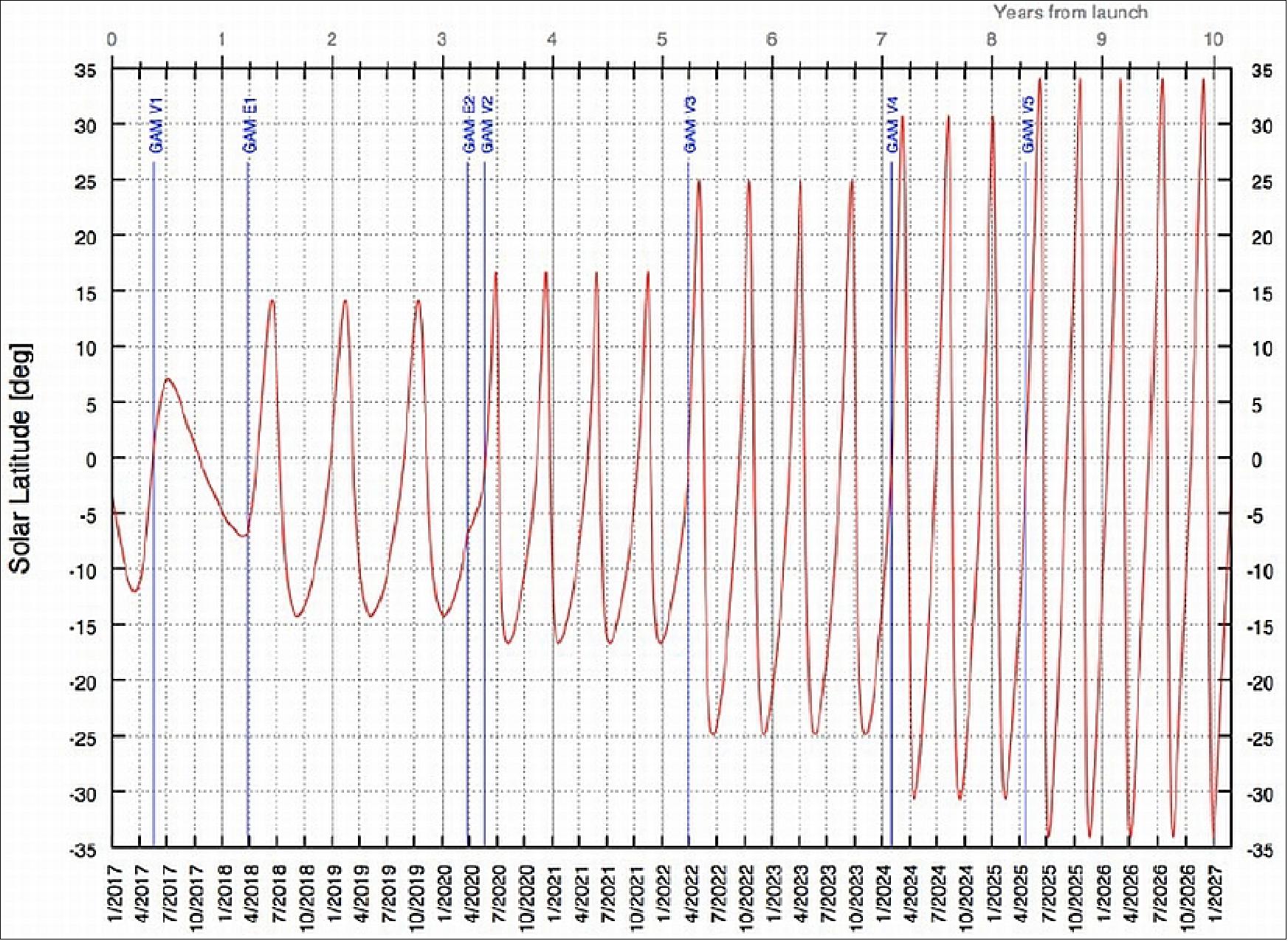
From its launch early in 2017, the Solar Orbiter will reach the nominal orbit around the Sun in 2020, operating in its near-Sun environment for at least 6 years, including the extended mission phase. During this period, the spacecraft will carry the science payload through 14 perihelion passages. At the same time, the heliocentric latitude will be gradually increased through repeated Venus gravity assist maneuvers, providing information about the behavior of the Sun at high latitudes.
Note: As of 25 March 2022, the previously single large SolO (Solar Orbiter) mission) file has been split into two files, to make the file handling manageable for all parties concerned, in particular for the user community.
• This article covers the SolO (Solar Orbiter) mission and its imagery in the period 2022 to 2020
Mission Status
• May 18, 2022: The Spectral Imaging of the Coronal Environment (SPICE) instrument on the ESA/NASA Solar Orbiter spacecraft records the composition of the corona, the Sun's outer atmosphere. 37)
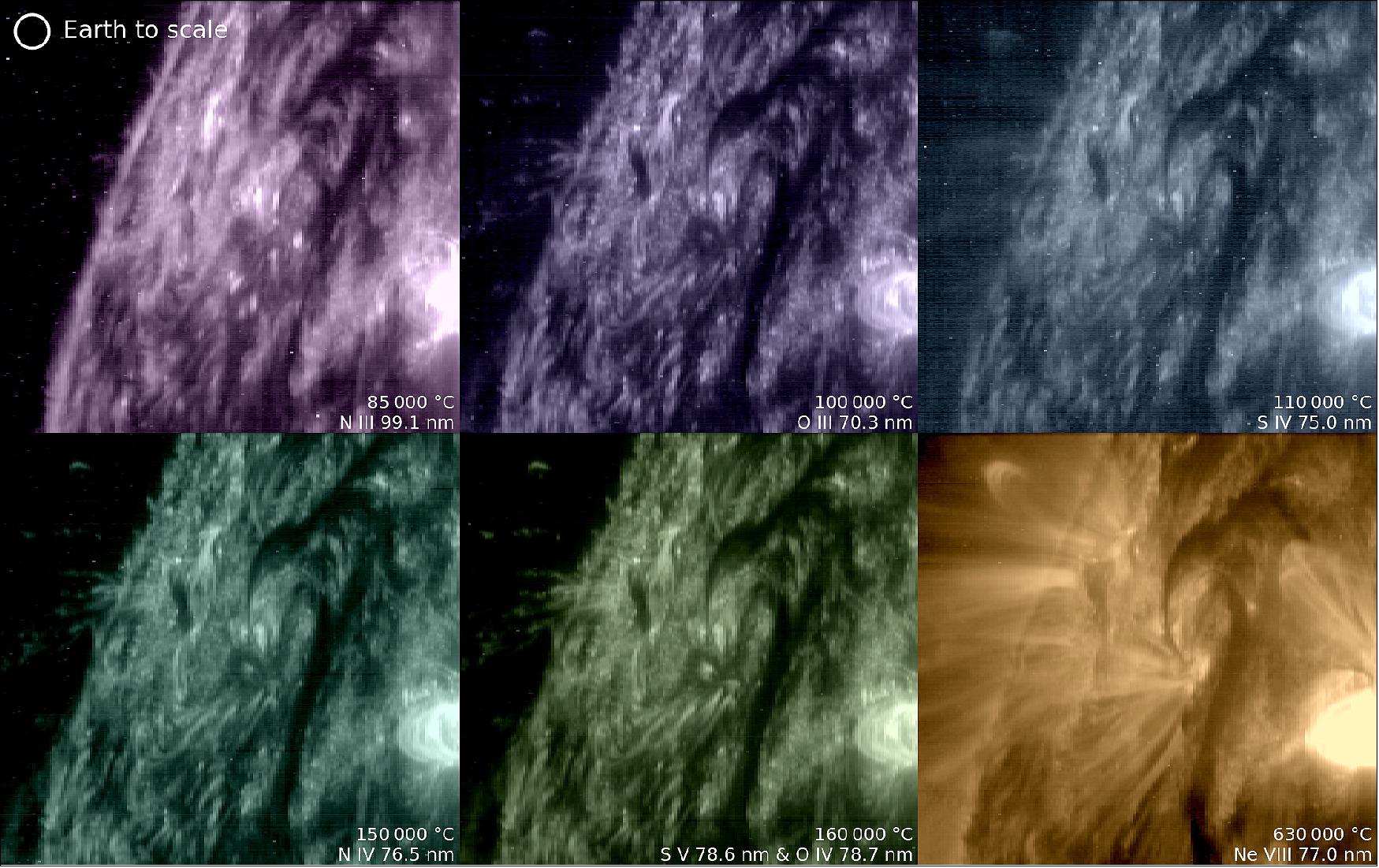
- The images reveal the composition of the Sun's atmosphere because each of these wavelengths is characteristically emitted by different species of atoms at different temperatures. Along the top row the 99 nanometres (nm) image shows nitrogen at 85,000°C; the 70 nm image shows oxygen at 100,000°C; and the 75 nm image shows sulphur at 110,000°C. Along the bottom row the 76.5 nm image shows nitrogen at 150,000°C, the 78 nm image shows both sulphur and oxygen at 160,000°C, and the 77 nm image shows neon at 630,000°C.
- The composition revealed by SPICE is compared to the composition of the solar wind recorded by Solar Orbiter's Solar Wind Analyser (SWA) instrument. This allows researchers to investigate the different possible acceleration mechanisms responsible for launching the solar wind.
• May 18, 2022: The Extreme Ultraviolet Imager (EUI) and the X-ray Spectrometer/Telescope (STIX) instruments aboard the ESA/NASA Solar Orbiter spacecraft captured a solar flare erupting from an active region on the face of the Sun on 2 March 2022. The EUI images show extreme ultraviolet light with a wavelength of 17 nanometers (174 Ångstroms) being emitted by solar atmospheric gasses with a temperature of around one million degrees Celsius. 38)
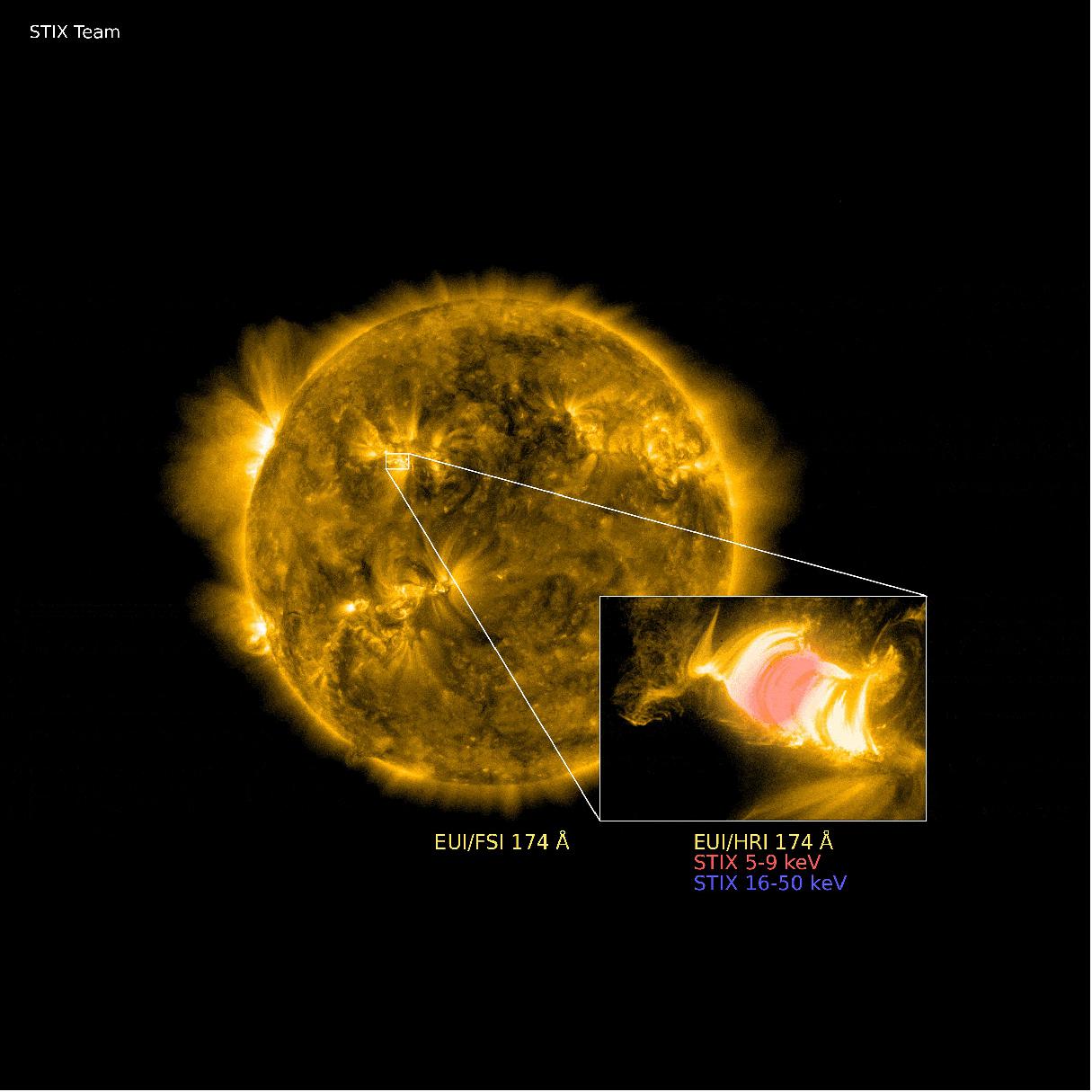
- EUI takes both full disc images using the Full Sun Imager (FSI) telescope, as well as detailed images of a smaller region using the High Resolution Imager (HRIEUV) telescope. The STIX detections have been overlaid on the zoomed-in EUI HRIEUV images. STIX records X-rays in two different energy bands. Lower energy X-rays are displayed in red, higher energy X-rays in blue. The flare emits mainly extreme ultraviolet light and lower energy X-rays but as it develops it also generates some higher energy X-rays too.
• May 18, 2022: Powerful flares, breathtaking views across the solar poles, and a curious solar ‘hedgehog' are amongst the haul of spectacular images, movies and data returned by Solar Orbiter from its first close approach to the Sun. Although the analysis of the new dataset has only just started, it is already clear that the ESA-led mission is providing the most extraordinary insights into the Sun's magnetic behaviour and the way this shapes space weather. 39)
- Solar Orbiter's closest approach to the Sun, known as perihelion, took place on 26 March. The spacecraft was inside the orbit of Mercury, at about one-third the distance from the Sun to the Earth, and its heatshield was reaching around 500°C. But it dissipated that heat with its innovative technology to keep the spacecraft safe and functioning.
- Solar Orbiter carries ten science instruments – nine are led by ESA Member States and one by NASA – all working together in close collaboration to provide unprecedented insight into how our local star ‘works'. Some are remote-sensing instruments that look at the Sun, while others are in-situ instruments that monitor the conditions around the spacecraft, enabling scientists to ‘join the dots' from what they see happening at the Sun, to what Solar Orbiter ‘feels' at its location in the solar wind millions of kilometres away.
- When it comes to perihelion, clearly the closer the spacecraft gets to the Sun, the finer the details the remote sensing instrument can see. And as luck would have it, the spacecraft also soaked up several solar flares and even an Earth-directed coronal mass ejection, providing a taste of real-time space weather forecasting, an endeavour that is becoming increasingly important because of the threat space weather poses to technology and astronauts.
• March 24, 2022: The ESA/NASA Solar Orbiter spacecraft is speeding towards its historic first close pass of the Sun, which happens midday on 26 March 2022. 40)
- In the days leading up to and around ‘Perihelion passage', teams at ESA have been working intensively on an observation campaign, and all ten instruments will be operating simultaneously to gather as much data as possible.
- This effort will include using its remote sensing instruments, like the Extreme Ultraviolet Imager (EUI) to image the Sun, as well as in-situ instruments to measure the solar wind as it flows past the spacecraft.
- Observing specific targets of scientific interest on the Sun requires close coordination between flight control teams and the flight dynamics experts at ESA's ESOC mission control centre, in Germany, and teams at the science operations centre at ESAC, in Spain.
- ESA teams are using the full-disc telescopes on board Solar Orbiter to identify dynamic activity – like moving sunspots – on the surface, then will use these specific locations to calculate accurate pointing of the narrow-angle imager for later detailed observation.
- ESA teams are using the full-disc telescopes on board Solar Orbiter to identify dynamic activity – like moving sunspots – on the surface, then will use these specific locations to calculate accurate pointing of the narrow-angle imager for later detailed observation.
- This cycle of using wide-angle images to select specific narrow-angle targets, then feeding the needed pointing back into flight control instructions takes place daily, with each iteration taking three days from initial imaging to uplink of new pointing instructions.
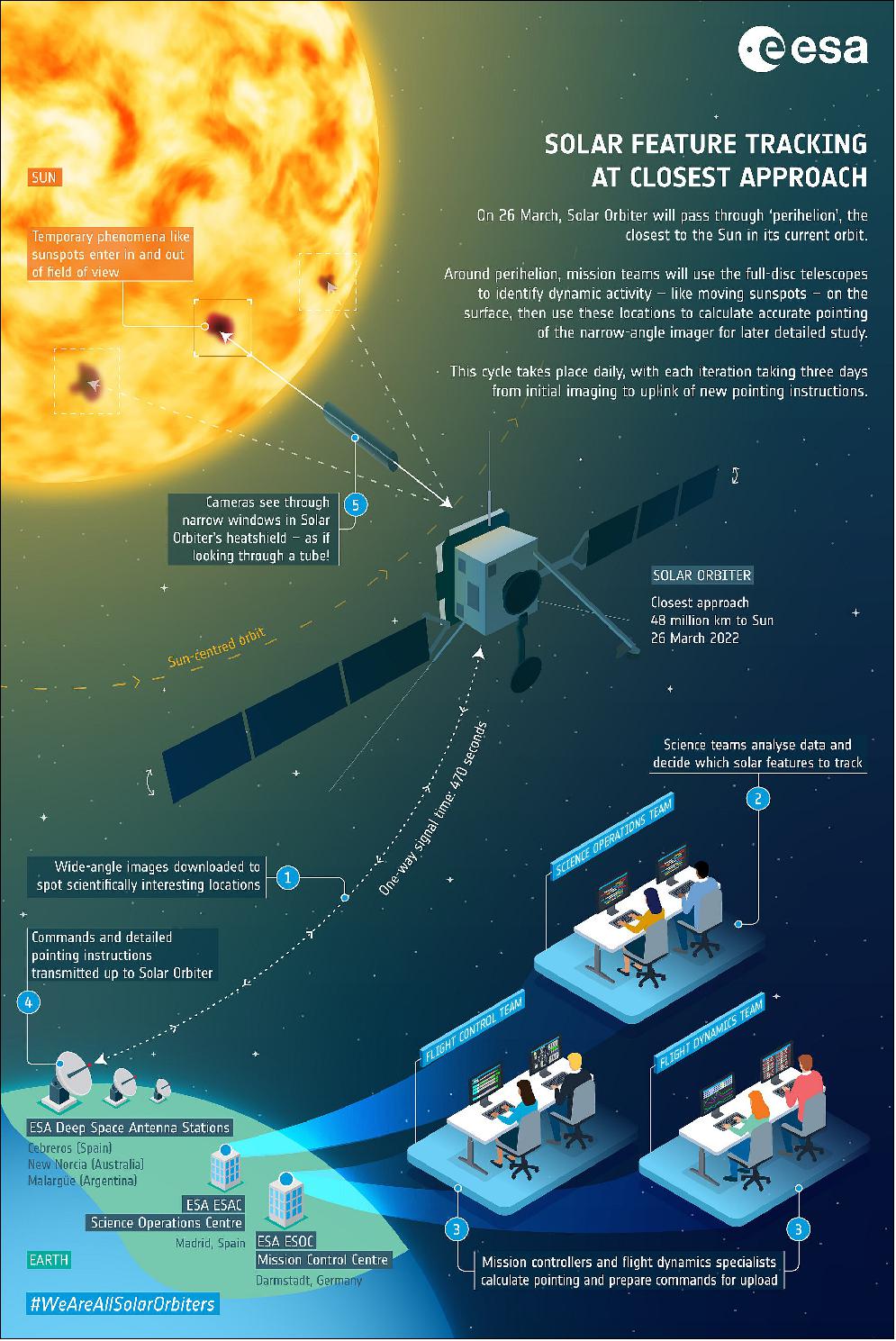
• March 24, 2022: Solar Orbiter's latest images shows the full Sun in unprecedented detail. They were taken on 7 March, when the spacecraft was crossing directly between the Earth and Sun. 41)
- One of the images (Figure 23), taken by the Extreme Ultraviolet Imager (EUI) is the highest resolution image of the Sun's full disc and outer atmosphere, the corona, ever taken.
- Another image, taken by the Spectral Imaging of the Coronal Environment (SPICE) instrument represents the first full Sun image of its kind in 50 years, and by far the best one, taken at the Lyman-beta wavelength of ultraviolet light that is emitted by hydrogen gas.
- The images were taken when Solar Orbiter was at a distance of roughly 75 million km, half way between our world and its parent star. The high-resolution telescope of EUI takes pictures of such high spatial resolution that, at that close distance, a mosaic of 25 individual images is needed to cover the entire Sun. Taken one after the other, the full image was captured over a period of more than four hours because each tile takes about 10 minutes, including the time for the spacecraft to point from one segment to the next.
- In total, the final image contains more than 83 million pixels in a 9148 x 9112 pixel grid. For comparison, this image has a resolution that is ten times better than what a 4k TV screen can display.
- EUI images the Sun at a wavelength of 17 nm, in the extreme ultraviolet region of the electromagnetic spectrum. This reveals the Sun's upper atmosphere, the corona, which has a temperature of around a million degrees Celsius.
- At the 2 o'clock (near the image of the Earth for scale) and 8 o'clock positions on the edges of the Sun, dark filaments can be seen projecting away from the surface. These ‘prominences' are prone to erupt, throwing huge quantities of coronal gas into space and creating ‘space weather' storms.
![Figure 23: The Sun as seen by Solar Orbiter in extreme ultraviolet light from a distance of roughly 75 million km. The image is a mosaic of 25 individual images taken on 7 March by the high resolution telescope of the Extreme Ultraviolet Imager (EUI) instrument. Taken at a wavelength of 17 nanometers, in the extreme ultraviolet region of the electromagnetic spectrum, this image reveals the Sun's upper atmosphere, the corona, which has a temperature of around a million degrees Celsius. In total, the final image contains more than 83 million pixels in a 9148 x 9112 pixel grid, making it the highest resolution image of the Sun's full disc and outer atmosphere, the corona, ever taken. - An image of Earth is also included for scale, at the 2 o'clock position [image credit: ESA & NASA/Solar Orbiter/EUI team; Data processing: E. Kraaikamp (ROB)]](https://eoportal.org/ftp/satellite-missions/s/SolO_200522/SolO_Auto4F.jpeg)
- In addition to EUI, the SPICE instrument was also recording data during the crossing. These too needed to be pieced together as a mosaic.
- SPICE is designed to trace the layers in the Sun's atmosphere from the corona, down to a layer known as the chromosphere, getting closer to the surface. The instrument does this by looking at the different wavelengths of extreme ultraviolet light that come from different atoms.
![Figure 24: Taking the Sun's temperature. Solar Orbiter took images of the Sun on 7 March, from a distance of roughly 75 million km, using its Spectral Imaging of the Coronal Environment (SPICE) instrument. SPICE takes simultaneous "spectral images" at several different wavelengths of the extreme ultraviolet spectrum by scanning its spectrometer slit across a region on the Sun. The different wavelengths recorded correspond to different layers in the Sun's lower atmosphere. Purple corresponds to hydrogen gas at a temperature of 10,000ºC, blue to carbon at 32,000ºC, green to oxygen at 320,000ºC, yellow to neon at 630,000ºC. Each full-Sun image is made up of a mosaic of 25 individual scans. It represents the best full Sun image taken at the Lyman beta wavelength of ultraviolet light that is emitted by hydrogen gas [ESA & NASA/Solar Orbiter/SPICE team; Data processing: G. Pelouze (IAS)]](https://eoportal.org/ftp/satellite-missions/s/SolO_200522/SolOTakingSun_s_temperature.gif)
- This will allow solar physicists to trace the extraordinarily powerful eruptions that take place in the corona down through the lower atmospheric layers. It will also allow them to study one of the most puzzling observations about the Sun: how the temperature is rising through the ascending atmospheric layers.
- Usually the temperature drops as you move away from a hot object. But above the Sun, the corona reaches a million degrees Celsius whereas the surface is only about 5000°C. Investigating this mystery is one of the key scientific objectives of Solar Orbiter.
- The images were taken on 7 March, precisely when Solar Orbiter crossed the Sun-Earth line, so the images can be compared with Earth-bound solar instruments and cross-calibrated. This will make it easier to compare results from different instruments and observatories in future.
![Figure 25: Solar Orbiter took images of the Sun on 7 March, from a distance of roughly 75 million km, using its Spectral Imaging of the Coronal Environment (SPICE) instrument. SPICE takes simultaneous "spectral images" at several different wavelengths of the extreme ultraviolet spectrum by scanning its spectrometer slit across a region on the Sun. The different wavelengths recorded correspond to different layers in the Sun's lower atmosphere. Purple corresponds to hydrogen gas at a temperature of 10,000ºC, blue to carbon at 32,000ºC, green to oxygen at 320,000ºC, yellow to neon at 630,000ºC. Each full-Sun image is made up of a mosaic of 25 individual scans. It represents the best full Sun image taken at the Lyman beta wavelength of ultraviolet light that is emitted by hydrogen gas[image credit: ESA & NASA/Solar Orbiter/SPICE team; Data processing: G. Pelouze (IAS)]](https://eoportal.org/ftp/satellite-missions/s/SolO_200522/SolO_Auto4E.jpeg)
- On 26 March, Solar Orbiter reaches another mission milestone: its first close perihelion. The spacecraft is now inside the orbit of Mercury, the inner planet, taking the highest resolution images of the Sun it can take. It is also recording data on the solar wind of particles that flows outwards from the Sun.
- And this is just the start, over the coming years the spacecraft will repeatedly fly this close to the Sun. It will also gradually raise its orientation to view the Sun's previously unobserved polar regions.
- Solar Orbiter is a partnership between ESA and NASA.
• March 7, 2022: The ESA/NASA Solar Orbiter spacecraft is speeding towards its historic first close pass of the Sun. On 14 March, the spacecraft will pass the orbit of Mercury, the scorched inner planet of our Solar System, and on 26 March it will reach closest approach to the Sun. 42)
- Today, Solar Orbiter is crossing directly between the Earth and the Sun, about halfway between our planet and its parent star, and this allows for a unique study of space weather and the Sun-Earth connection.
- The Sun releases a constant stream of particles into space. This is known as the solar wind. It carries the Sun's magnetic field into space, where it can interact with planets to create aurorae and disrupt electrical technology. Magnetic activity on the Sun, often taking place above sunspots, can create gusts in the wind enhancing these effects.
- This behaviour is known as space weather, and scientists can use today's Earth-Sun line crossing to study it in a unique way. They will combine Solar Orbiter observations with those of other spacecraft operating nearer the Earth, such as the Hinode and IRIS spacecraft in Earth orbit, and SOHO, stationed 1.5 million kilometres away from Earth. This will allow them to join the dots of any space weather event as it crosses the 150 million kilometres between the Sun and the Earth.
- Solar Orbiter's remote sensing instruments may also be able to pinpoint the origin of any event on the solar surface. Such ‘linkage science' is one of the main drivers behind the Solar Orbiter mission. Even if no big event takes place there is still a lot of science that can be performed in analysing the evolution of the same packet of solar wind as it travels outwards into the Solar System.
- Solar Orbiter's remote sensing instruments may also be able to pinpoint the origin of any event on the solar surface. Such ‘linkage science' is one of the main drivers behind the Solar Orbiter mission. Even if no big event takes place there is still a lot of science that can be performed in analysing the evolution of the same packet of solar wind as it travels outwards into the Solar System.
- On 10 February, ESA renamed its upcoming space weather mission from Lagrange to ESA Vigil. Launching sometime in the middle of the decade, the spacecraft will be a solar watchdog, constantly monitoring the Sun for unpredictable magnetic activity so that Earth's infrastructure, satellites, inhabitants and space explorers can be protected from these unpredictable events.
- Solar Orbiter is currently around 75 million kilometres away from the Sun. This is the same distance as the spacecraft achieved during its close pass to the Sun on 15 June 2020 but nothing compared to how close it will now get.
- "From this point onwards, we are ‘entering the unknown' as far as Solar Orbiter's observations of the Sun are concerned," says Daniel Müller, Solar Orbiter Project Scientist.
• February 18, 2022: The ESA/NASA Solar Orbiter spacecraft has captured the largest solar prominence eruption ever observed in a single image together with the full solar disc. 43)
- Solar prominences are large structures of tangled magnetic field lines that keep dense concentrations of solar plasma suspended above the Sun's surface, sometimes taking the form of arching loops. They are often associated with coronal mass ejections, which if directed towards Earth, can wreak havoc with our technology and everyday lives.
- This latest event took place on 15 February and extended millions of kilometres into space. The coronal mass ejection was not directed at Earth. In fact, it is travelling away from us. There is no signature of the eruption on the solar disc facing the spacecraft – which is currently approaching the Earth-Sun line – meaning that it must have originated from the side of the Sun facing away from us.
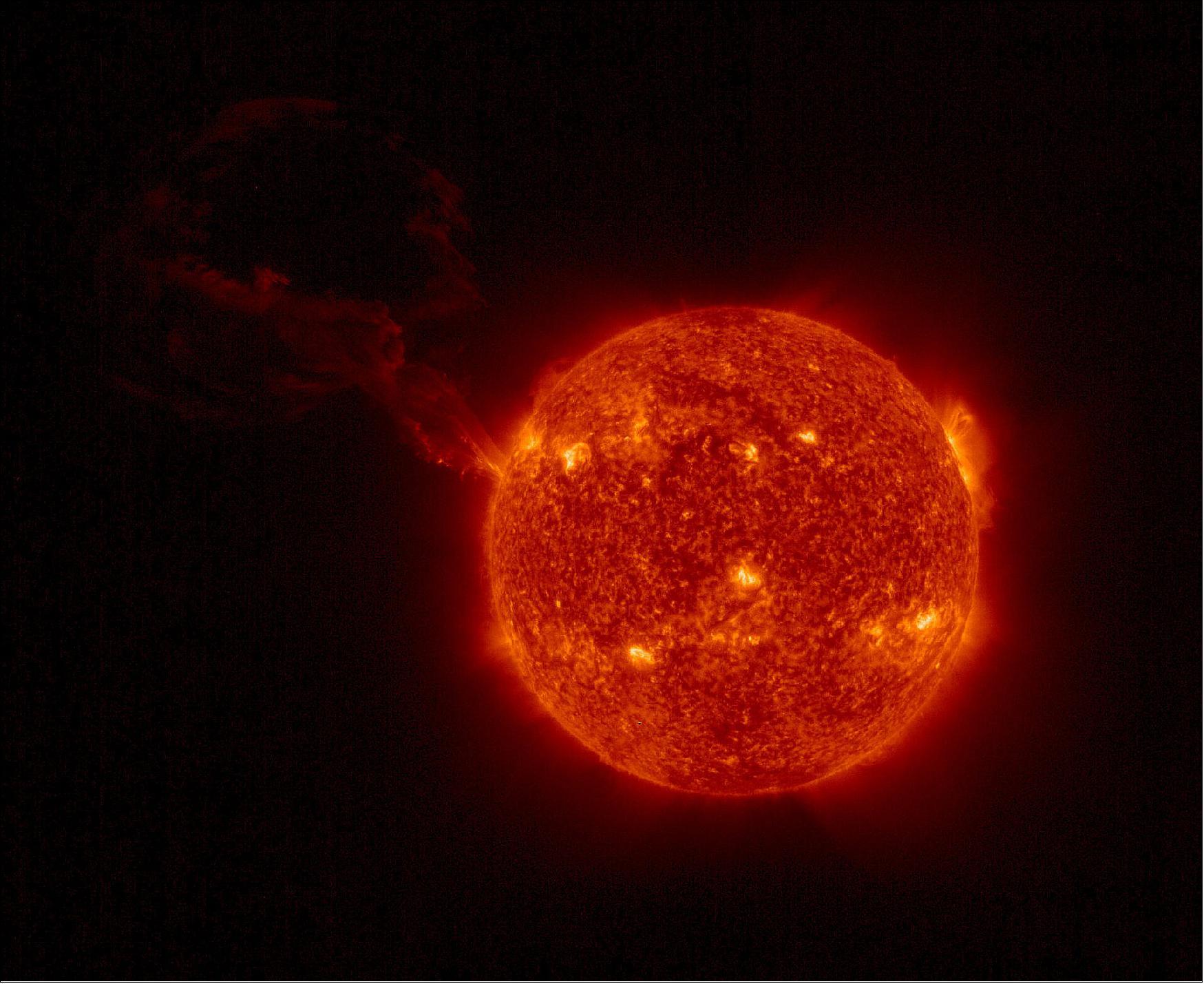
• January 25, 2022: For the second time in its mission so far, the ESA/NASA Solar Orbiter spacecraft has flown through the tail of a comet. Predicted in advance by astronomers at University College London, UK, the spacecraft collected a wealth of science data that now awaits full analysis. 44)
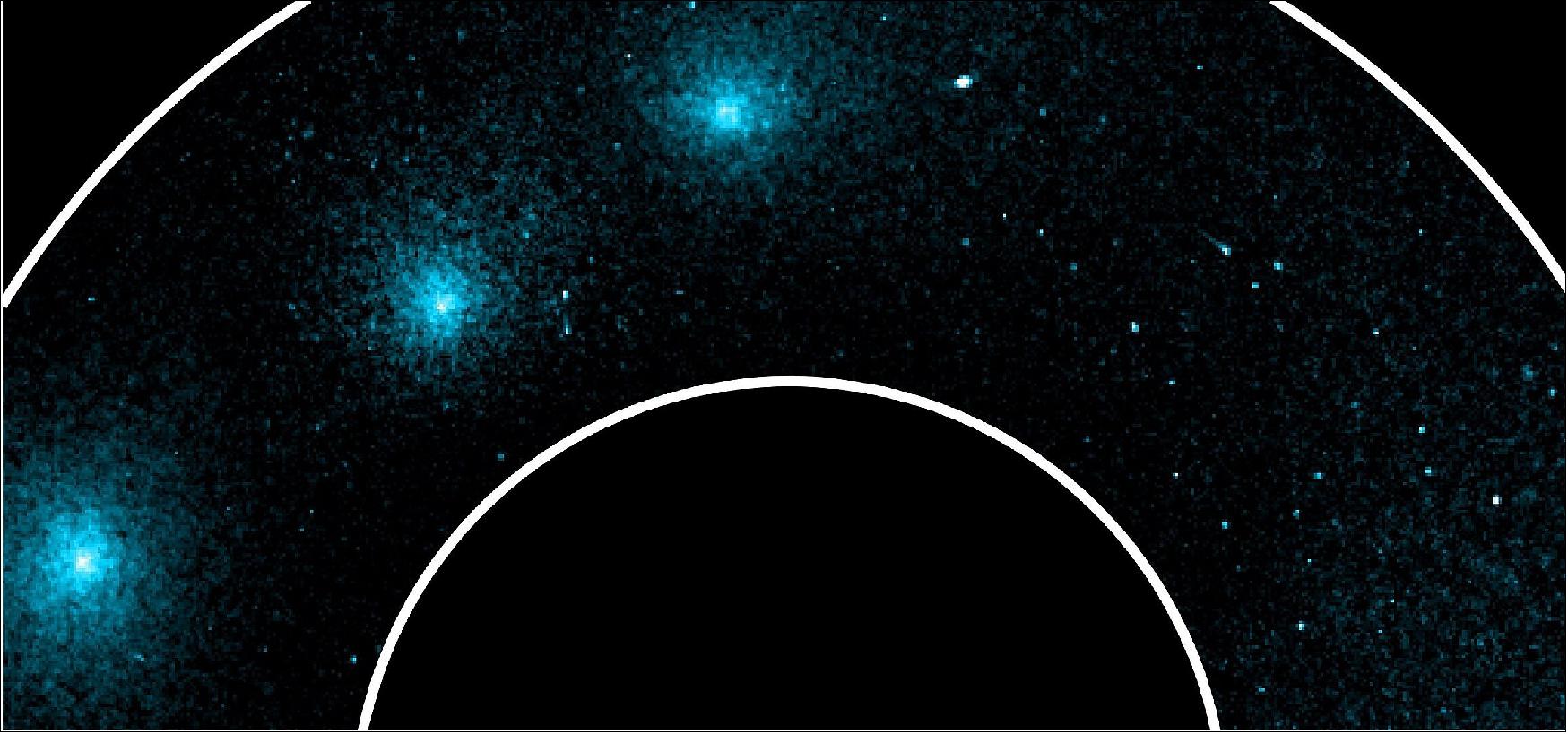
- For a spacecraft designed to conduct unique studies of the Sun, Solar Orbiter is also making a name for itself exploring comets. For several days centered on 1200-1300 UT on 17 December 2021, the spacecraft found itself flying through the tail of Comet C/2021 A1 Leonard.
- The encounter captured information about the particles and magnetic field present in the tail of the comet. This will allow astronomers to study the way the comet interacts with the solar wind, a variable wind of particles and magnetic field that emanate from the Sun and sweep through the solar system.
- The crossing had been predicted by Samuel Grant, a post graduate student at University College London's Mullard Space Science Laboratory. He adapted an existing computer program that compared spacecraft orbits with comet orbits to include the effects of the solar wind and its ability to shape a comet's tail.
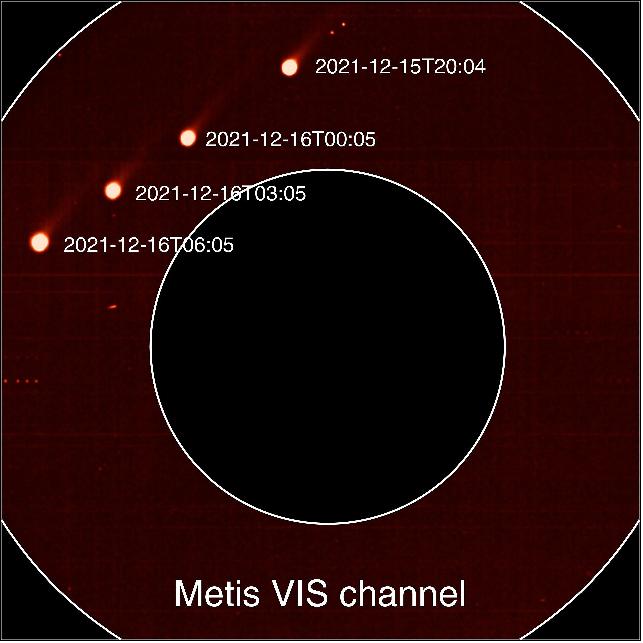
- "I ran it with Comet Leonard and Solar Orbiter with a few guesses for the speed of the solar wind. And that's when I saw that even for quite a wide range of solar wind speeds it seemed like there would be a crossing," he says.

- The plot illustrates the accumulation of ‘hits' of different cometary and solar particles over the course of each day, and records the time it takes for individual particles to travel through the core of the instrument (time of flight), along with its energy/charge. In general, heavier particles take longer to travel than lighter ones.
- Particles of different mass/charge distribute along specific lines in the plot. The regular solar wind particles cluster along one line (labelled Vsw) in high numbers (indicated by the red and green colours). Boundary lines are also marked for particles travelling twice as fast as solar wind particles (2Vsw) and 0.8 times as fast (0.8Vsw). The density of green colours in distinct bands in the right hand side of the plot reveals particles that have different charge characteristics to those expected in the solar wind, and, in addition, molecules simply not found in the solar wind.
- Data like this collected over many days helps profile the comet's ion tail. The data are used to determine the rate at which the solar wind is stripping material from the comet, for example by comparing the abundances of ‘parent' molecules (e.g. carbon monoxide, CO), with the individual atomic components (in that case, carbon and oxygen). Combined with magnetic field data, scientists will also be able to explore local space plasma instabilities generated by the interaction of the newly created ions with the solar wind itself.
- At the time of the crossing, Solar Orbiter was relatively close to the Earth having passed by on 27 November 2021 for a gravity assist maneuver that marked the beginning of the mission's science phase, and placed the spacecraft on course for its March 2022 close approach to the Sun. The comet's nucleus was 44.5 million km away, near to the planet Venus, but its giant tail stretched across space to Earth's orbit and beyond.
- So far, the best detection of the comet's tail from Solar Orbiter has come from the Solar Wind Analyser (SWA) instrument suite. Its Heavy Ion Sensor (HIS) clearly measured atoms, ions and even molecules that are attributable to the comet rather than the solar wind.
- Ions are atoms or molecules that have been stripped of one or more electron and now carry a net positive electrical charge. SWA-HIS detected ions of oxygen, carbon, molecular nitrogen, and molecules of carbon monoxide, carbon dioxide and possibly water. "Because of their small charge, these ions are all clearly of cometary origin," says Stefano Livi, Lead Investigator of SWA-HIS from SwRI (Southwest Research Institute), Texas.
![Figure 32: This data plot uses solar wind speed and direction data from the Solar Wind Analyser's proton and alpha sensor (SWA-PAS) to estimate how close the ESA/NASA Solar Orbiter spacecraft approached to the centre of Comet C/2021 A1 Leonard's ion tail during December 2021. The plot records how close each packet of solar wind detected by SWA-PAS is thought to have got to the comet's nucleus during its journey from the Sun to the spacecraft. The left axis gives the scale in astronomical units (au), where 1 au is the distance from the Sun to the Earth, and the same distance is shown in kilometres on the right axis. - Changes in the solar wind flow speed and direction are responsible for the variations in the plotted distance. There are short data acquisition gaps on 15 and 17 December [image credit: ESA/Solar Orbiter/SWA team & S. Grant (UCL)]](https://eoportal.org/ftp/satellite-missions/s/SolO_200522/SolO_Auto4A.jpeg)
- As a comet moves through space, it tends to drape the Sun's magnetic field around it. This magnetic field is being carried by the solar wind, and the draping creates discontinuities where the polarity of the magnetic field changes sharply from north to south and vice versa.
- The magnetometer instrument (MAG) data does indeed suggest the presence of such draped magnetic field structures but there is more analysis to be done to be absolutely sure. "We are in the process of investigating some smaller scale magnetic perturbations seen in our data and combining them with measurements from Solar Orbiter's particle sensors to understand their possible cometary origin," says Lorenzo Matteini, a co-investigator on MAG from Imperial College, London.
- In addition to the particle data, Solar Orbiter also acquired images.
- METIS is Solar Orbiter's multi-wavelength coronagraph. It can perform ultraviolet observations that see the Lyman alpha emission given out by hydrogen, and it can measure the polarisation of visible light. During 15 and 16 December it captured the distant head of the comet simultaneously in both visible and ultraviolet light. These images are now being analysed by the instrument team. "The visible light images can hint at the rate at which the comet is ejecting dust, while the ultraviolet images can give the water production rate," says Alain Corso, a METIS co-investigator at the CNR-Istituto di Fotonica e Nanotecnologie, Padova, Italy.
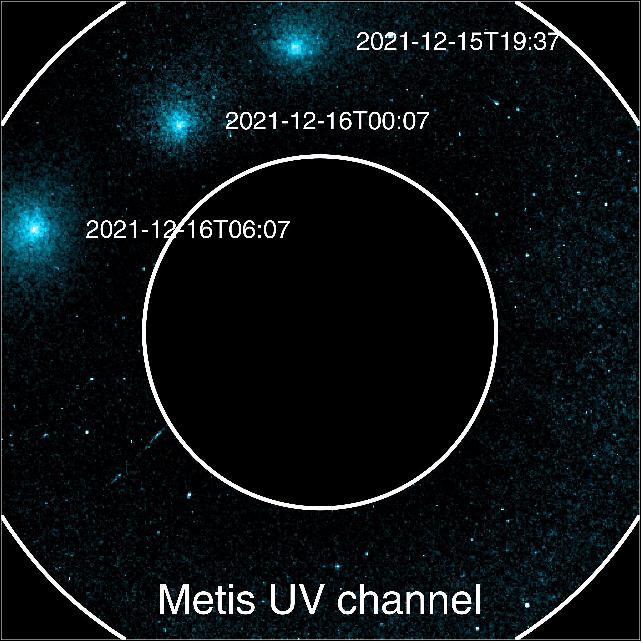
- The Solar Orbiter Heliospheric Imager (SoloHI) also captured data. These images show large parts of the comet's ion tail taken while the spacecraft itself was inside the tail. As the image sequence progresses, changes in the tail can be seen in response to variations in the solar wind speed and direction.
- And it was not just Solar Orbiter that was watching the crossing. The ESA/NASA SOHO mission and NASA's STEREO-A and Parker Solar Probe spacecraft were observing from afar. This means that not only do astronomers now have data from inside the tail, they also have contextual images from these other spacecraft.
- Comet tail crossings are relatively rare events. Of those that have been detected, most have been noticed only after the event. The ESA/NASA Ulysses mission encountered three comet ion tails, including that of C/1996 B2 Hyakutake in May 1996, and C/2006 P1 McNaught in early 2007. Solar Orbiter itself crossed the tail of fragmenting comet C/2019 Y4 ATLAS in May and June 2020, shortly after launching.
![Figure 34: The ESA/NASA Solar Orbiter spacecraft flew through the tail of Comet C/2021 A1 Leonard in December 2021, collecting images and in-situ solar wind and particle data. At the same time, SOHO (ESA/NASA), Parker Solar Probe (NASA) and STEREO-A (NASA) were also watching the comet's evolution from other angles. The graphic shows the approximate relative positions of the planets, comet and spacecraft on 17 December 2021 and is not to scale. Very approximate fields of view are indicated for selected instruments: SoloHI on Solar Orbiter and SECCHI on STEREO-A [image credit: G. Jones & S. Grant (UCL)]](https://eoportal.org/ftp/satellite-missions/s/SolO_200522/SolO_Auto48.jpeg)
- Whereas the early crossings were a surprise, both of Solar Orbiter's encounters were predicted in advance thanks to the computer code developed by Geraint Jones, University College London Mullard Space Science Laboratory, and extended by Samuel.
- "The big advantage is that for basically no effort on the spacecraft's part, you get to sample a comet at a massive distance. That's pretty exciting," says Samuel, who is now looking at archive data from other spacecraft looking for comet tail crossings that have so far gone unnoticed.
- The work also helps build experience for ESA's Comet Interceptor mission, for which Geraint is the Science Team Lead. The mission will visit an as-yet undiscovered comet, making a flyby of the target with three spacecraft to create a 3D profile of a 'dynamically new' object that contains unprocessed material surviving from the dawn of the Solar System.
- In the meantime, the instrument teams on Solar Orbiter are busy analysing the Comet Leonard data not only for what it can tell them about the comet but about the solar wind as well.
- "This kind of additional science is always an exciting part of a space mission," says Daniel Müller, ESA Project Scientist for Solar Orbiter. "When the comet ATLAS crossing was predicted, we were still calibrating the spacecraft and its instruments. Also, the comet fragmented just before we got there. But with Comet Leonard we were totally ready – and the comet didn't fall apart."
- In March, Solar Orbiter make its closest pass to the Sun yet at a distance of 0.32 au (approximately one-third of the Earth-Sun distance, or about 50 million km). It is one of almost 20 close passes to the Sun that will occur during the next decade. These will result in unprecedented images and data, not only from close up, but also from the Sun's never-before seen polar regions.
- "There is so much to look forward to with Solar Orbiter, we're only just getting started," says Daniel.
• December 21, 2021: Comet Leonard, a mass of space dust, rock and ice about a kilometer across is heading for a close pass of the Sun on 3 January, and the ESA/NASA Solar Orbiter spacecraft has been watching its evolution over the last days. 45)
- The comet is currently on its inbound journey around the Sun with its tail streaking out behind. When SoloHI recorded these images, the comet was approximately between the Sun and the spacecraft, with its gas and dust tails pointing towards the spacecraft. Toward the end of the image sequence, our view of both of the tails improves as the viewing angle at which we see the comet increases, and SoloHI gets a side-on view of the comet.
- A faint coronal mass ejection front is also visible moving from the right hand side of the frame in the final second of the movie.
- SoloHI will continue observing the comet until it leaves its field of view on 22 December, and will be complemented by other instrument observations.
- Ground-based telescopes and other spacecraft have also been following the comet on its journey through the Solar System and providing images, including NASA's Solar Terrestrial Relations Observatory-A spacecraft – see here.
- Comet Leonard, formally known as C/2021 A1 (Leonard), was discovered in January 2021 by Gregory Leonard, who spotted it in images taken from the Mt. Lemmon Observatory in Arizona. Its closest pass on 3 January 2022 will take it within 90 million kilometers of the Sun, slightly more than half Earth's distance to the Sun. If it doesn't disintegrate, its trajectory will fling it into interstellar space, never to return.
• December 14, 2021: For a mission yet to have entered its main science phase, Solar Orbiter has already generated a lot of great science. Today sees the publication of a wealth of results from the mission's cruise phase. 46)
- Forensic observations of the solar surface, measurements of a giant outburst of energetic particles, and an encounter with a comet's tail are just some of the highlights out of the more than fifty papers comprising a special issue of Astronomy and Astrophysics and presented today at the annual AGU meeting.
- "The results published today demonstrate the variety of solar science that the mission is making possible, and signals the wealth of data that is now flowing back to Earth," says Yannis Zouganelis, ESA Deputy Project Scientist for Solar Orbiter.
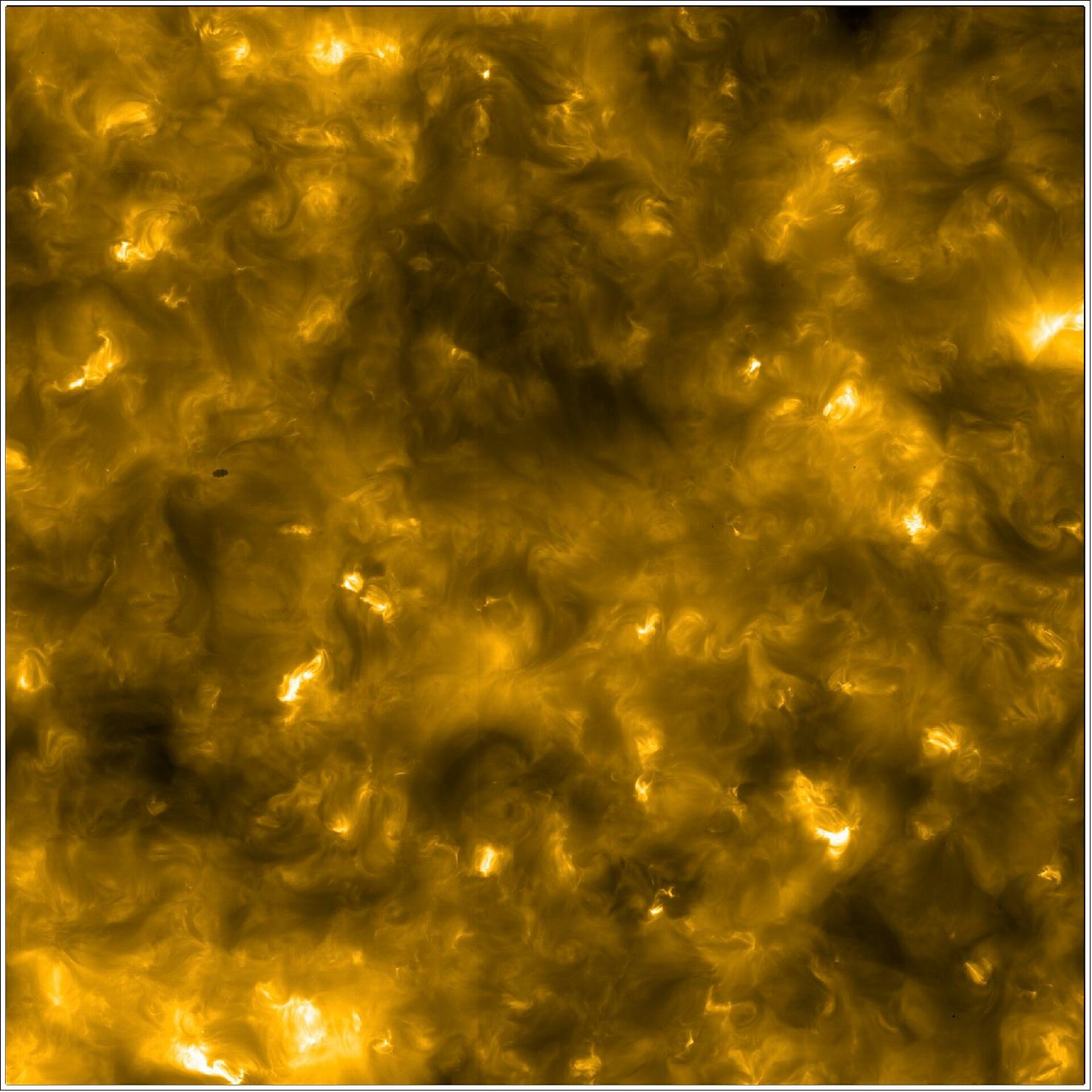
- Solar Orbiter's cruise phase began on 15 June 2020, and lasted until 27 November 2021. During that time, the spacecraft acquired scientific data with its in-situ instruments, which are designed to measure the environment around the spacecraft. It also used its remote sensing equipment to look at the Sun in order to characterise and calibrate those instruments. Some of these data turned out to be of such good quality that they enabled the first scientific studies to be undertaken ahead of the main science phase, which began in late November 2021.
Solar Orbiter's First Widespread Energetic Particle Event
- As well as the ‘small-scale' campfires, Solar Orbiter has also witnessed its first large-scale event. On 29 November 2020, the first widespread energetic particle event for several years burst from the Sun.
- The Sun goes through a cycle of magnetic activity that lasts approximately 11 years, and this particular event was the first widespread energetic particle event of cycle 25. As the name implies, the event spread particles across a large swathe of the inner solar system. By the time the eruption had reached Earth's distance, the ejected particles were spread over more than 230 degrees of solar longitude.
- They were detected not only by Solar Orbiter, but also by NASA's Parker Solar Probe and STEREO-A, and the ESA/NASA SOHO spacecraft, all of which were close to Earth's orbit but at varying solar longitudes. So, the question is how big was the event's source region on the Sun, and how much did the eruption expand after it was released? This is where Solar Orbiter's goal of ‘linkage science' becomes important.
- "I come from the in-situ observations," says Alexander Kolhoff, Institut für Experimentelle und Angewandte Physik, Christian-Albrechts-Universität zu Kiel, Germany, who led the analysis of the November event. "We see a particle event around the spacecraft and then go to the remote sensing observations and try to pinpoint the source on the Sun."
- In this particular case, the data is inconclusive about whether the size of the source region alone was large enough to explain the wide spread of particles or not. But the hints in the data are enough to show great promise as the scientists continue to refine this technique.
Tracking Down the Solar Stealth CMEs
- Also making painstakingly detailed observations of the solar surface was Jennifer O'Kane, Mullard Space Science Laboratory, University College London, UK. Together with colleagues, she went in search of so-called Stealth CMEs.
- CME stands for coronal mass ejection. These are the giant eruptions of solar plasma and magnetic field that usually occur alongside solar flares — an explosive magnetic event in the Sun's lower atmosphere that ejects the particles out into space. In the case of a stealth CME, however, there doesn't appear to be an associated flare.
- Using the most sophisticated image processing tools available, Jennifer looked at solar images to see if she could find evidence of a triggering event that launched a CME in April 2020.
- Its magnetic field strength, as measured by Solar Orbiter, was particularly large as well, around double that of a normal CME, but the puzzle was that the visible surface of the Sun was completely blank at that time. There were no sunspots or any other active regions. It was only the high magnetic field strength of the plasma that engulfed Solar Orbiter that alerted the team to the CME in the first place.
- After a painstaking search of the data, Jennifer found a dark region in the extreme ultraviolet images that indicated a low-density cavity in the solar corona, that lifted off very slowly from the Sun.
- Slow in this context is another relative term. Whereas most CMEs travel at hundreds or even thousands of kilometres a second, this one was moving outwards at tens of kilometres per second.
- It was the most difficult event that I've ever studied," says Jennifer, referring to how much effort it took to find even a hint of its origin.
- From a space weather forecasting perspective, stealth CMEs are a particular challenge because forecasters rely on seeing something on the Sun that they can recognise in real time in order to know that something is incoming that might change the near-Earth space environment.
Rendezvous with a Comet's Tail
- Lorenzo Matteini, Imperial College London, UK, led another painstaking investigation to determine whether Solar Orbiter has crossed the tail of Comet ATLAS during June 2020.
- The possible crossing was predicted shortly after Solar Orbiter's launch and so the team scrambled to make sure at least some instruments were ready in time to acquire data. By a rather cruel twist of fate, however, just ten days before the crossing, the comet disintegrated under the heat of the Sun and the beautiful tail faded.
- Nevertheless, Lorenzo and his colleagues found evidence consistent with a crossing of the comet's tail remnant in data taken on 4 June. Specifically, they saw the magnetic field around Solar Orbiter suddenly change its polarity, which would be expected if the Sun's magnetic field were draped around a piece of the broken comet's nucleus.
- "This is the first time that we have encountered a comet tail inside Earth's orbit," says Lorenzo.
- And it may not be the last. Comets are falling in towards the Sun all the time. The way they interact with the Sun's magnetic field provides yet another way for Solar Orbiter to investigate this fascinating region of the solar system.
- Following its November 2021 flyby of Earth, Solar Orbiter is now in its main science phase. All involved are preparing for its close pass of the Sun in March 2022.
- "I couldn't be more pleased with the mission. These results show both how much great science has already been done, and how much there is still to come," says Daniel Müller, ESA Project Scientist for Solar Orbiter.
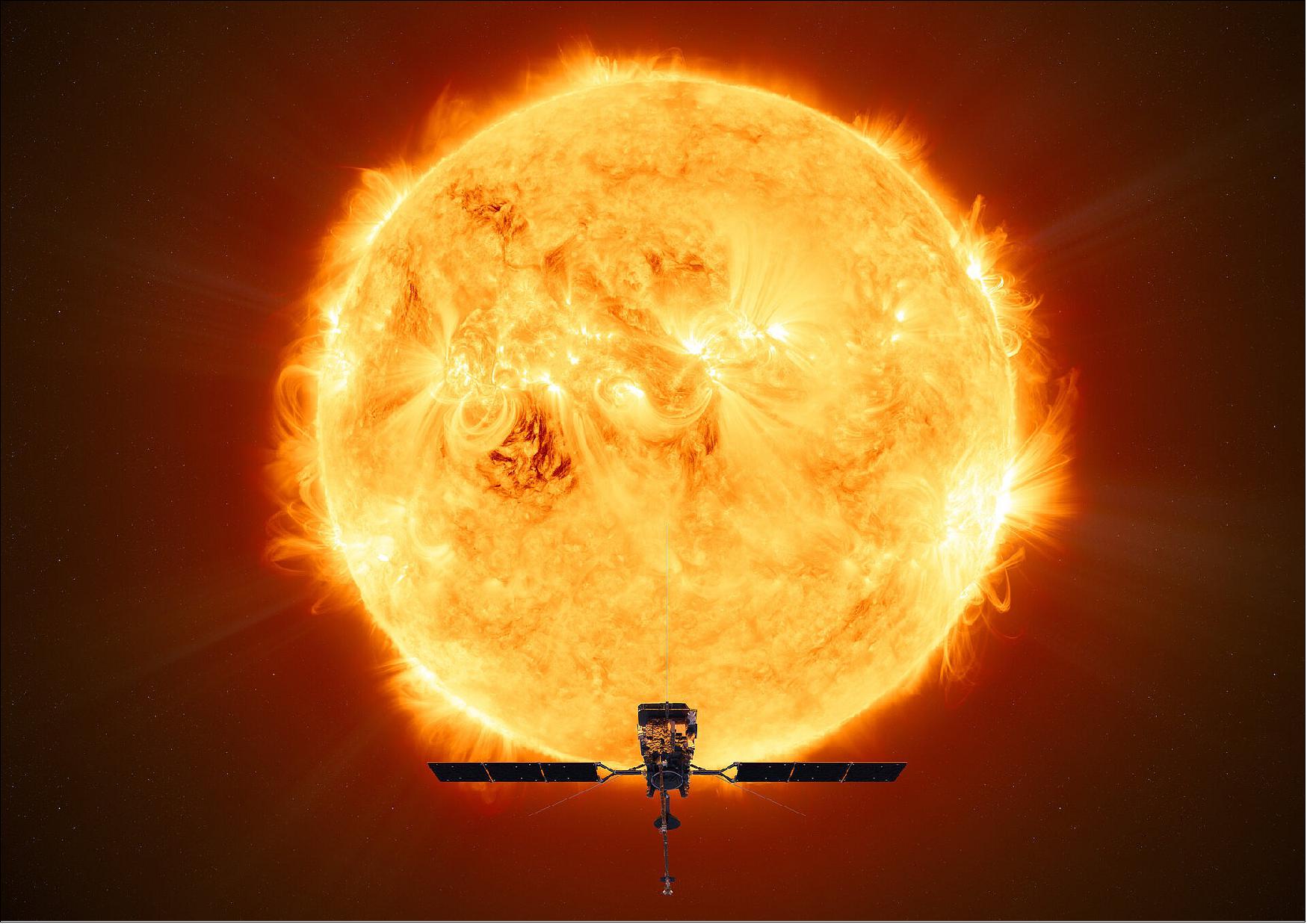
• November 18, 2021: Solar Orbiter is returning to Earth for a flyby before starting its main science mission to explore the Sun and its connection to ‘space weather'. During the flyby Solar Orbiter must pass through the clouds of space debris that surround our planet, making this maneuver the riskiest flyby yet for a science mission. 47)

Navigating Risk
- Solar Orbiter's Earth flyby takes place on 27 November. At 04:30 GMT (05:30 CET) on that day, the spacecraft will be at its closest approach, just 460 km above North Africa and the Canary Islands. This is almost as close as the orbit of the International Space Station.
- The maneuver is essential to decrease the energy of the spacecraft and line it up for its next close pass of the Sun but it comes with a risk. The spacecraft must pass through two orbital regions, each of which is populated with space debris.
- The first is the geostationary ring of satellites at 36,000 km, and the second is the collection of low Earth orbits at around 400 km. As a result, there is a small risk of a collision. Solar Orbiter's operations team are monitoring the situation very closely and will alter the spacecraft's trajectory if it appears to be in any danger.
Earth Science Opportunity
- On the plus side, the flyby offers a unique opportunity to study the Earth's magnetic field. This is a subject of intense interest because the magnetic field is our atmosphere's interface with the solar wind, the constant ‘wind' of particles given off by the Sun. Not only can particles from the solar wind penetrate the magnetic field and spark the aurora in our skies, but atoms from our atmosphere can also be lost into space.
- The details of these interactions are being studied by two ESA missions: Cluster's four satellites at 60,000 km in altitude and Swarm's three spacecraft at 400 km. Multiple spacecraft are needed to break the so-called space-time ambiguity. This is the name given to the uncertainty over whether a change has taken place because a spacecraft has flown into a different region with different conditions (a change in space) or is flying through a region that changes its conditions (a change in time).
- Solar Orbiter's flyby offers a unique opportunity to take even more data. It will sweep into the Earth's magnetic field from out beyond Clusters orbit, approach Swarm's orbit at closest approach and then fly back out again. This will provide even more data points from which to reconstruct the condition and behavior of Earth's magnetic field during the flyby.
- "This flyby is exciting: seeing what Solar Orbiter sees in our part of space, and how that compares to what we are seeing, and if there are surprises, what are they?" says Anja Strømme, Swarm Mission Manager.
Cruise Phase Complete
- The flyby marks a major milestone for Solar Orbiter. From its launch in February 2020 to July of that year, the spacecraft was in its commissioning phase, during which the scientists and engineers tested out the spacecraft and its instruments. From July 2020 to now, Solar Orbiter has been in the cruise phase. During this time, the in-situ instruments have been taking measurements of the solar wind and other conditions around the spacecraft, while the remote sensing instruments designed to look at the Sun have been in their extended calibration and characterization mode.
- Despite Solar Orbiter not yet being in full science mode, a lot of science has been produced.
- "Scientifically, this exceeded our expectations by a large margin," says Daniel Müller, Solar Orbiter Project Scientist. He explains that an upgrade to the ESA Ground Station Network allowed Solar Orbiter to send more data than expected back to Earth, and the mission's scientists have been quick to take advantage. More than fifty papers detailing Solar Orbiter's cruise phase science results are to be published in December by the journal Astronomy & Astrophysics.
Closer to the Sun
- Now, however, it is time to start operating the two sets of instruments together as the mission shifts into the main science phase, and the anticipation is palpable. In March, Solar Orbiter will make a close pass to the Sun, called perihelion. Its first perihelion took place in June 2020, with the spacecraft closing to 77 million km. This time, Solar Orbiter will draw to within 50 million km – providing a significant boost to the science that can be done.
- "This will be at a third of the distance between the Sun and Earth. So compared to all the interesting high resolution images that we've already gotten everything now will be zoomed in by about a factor of two," says Daniel.
- This includes new views of the enigmatic ‘campfires' that Solar Orbiter saw at the first perihelion. The campfires could hold clues about how the Sun's outer atmosphere has a temperature of millions of degrees, while the surface has a temperature of thousands – which seemingly defies physics because heat should not be able to flow from a colder to a hotter object.
- And while Solar Orbiter is not going as close to the Sun as NASA's Parker Solar Probe, this is by design because it allows Solar Orbiter to not only measure what is happening in the solar wind, but to also carry telescopes that can look at the Sun without being destroyed by the heat. The two data sets can then be compared to link activity on the Sun's surface to the space weather around the spacecraft.
- "This linkage science is what I find most exciting," says Yannis Zouganelis, Solar Orbiter Deputy Project Scientist.
Observing Challenge
- But before any of this takes place, Solar Orbiter must complete its flyby of Earth. And this presents an opportunity for eagle-eyed sky watchers to bid a final farewell to the spacecraft before it heads forever into deep space.
- In the moments leading up to closest approach, skywatchers in the Canaries and North Africa could catch a brief glimpse of the spacecraft speeding through the sky. It will be travelling at about 0.3 degrees per second, which is just over half the apparent diameter of the Moon every second. For most observers it will be too faint to spot with the unaided eye, and too fast for telescopes to track, so binoculars should provide the best chance of catching a glimpse.
- When Solar Orbiter re-emerges from the Earth's shadow it will be on course for its rendezvous with the Sun and the never-before-seen solar polar regions. The science phase of this ambition mission will have begun.

- Solar Orbiter will concentrate on four main areas of investigation; very broadly:
a) Solar wind: What drives the solar wind and the acceleration of solar wind particles?
b) Polar regions: What happens in the polar regions when the solar magnetic field flips polarity?
c) Magnetic field: How is magnetic field generated inside the Sun and how does it propagate through the Sun's atmosphere and outwards into space?
d) Space weather: How do sudden events like flares and coronal mass ejections impact the Solar System, and how do solar eruptions produce the energetic particles that lead to extreme space weather at Earth?
- Solar Orbiter is a space mission of international collaboration between ESA and NASA.
• August 9, 2021: On Earth, fuel is a limited, precious resource. In space, we trade energy freely with the planets. 48)
- As Arthur C. Clarke said in 1987 "Nature always balances her books", referring to the fact that energy in the universe is always conserved: it cannot appear from nowhere or disappear into nothing.
- As such, flying spacecraft through the Solar System is about the clever management of energy – stealing or giving to passing planets depending on where we want to go.
- This transfer of energy is called a gravity assist, slingshot, planetary swingby or flyby, and without them we could not have voyaged to the many corners of the Solar System we have today.
With Orbits, Size Matters
- In space, "speeding up" or "slowing down" only make sense with respect to another object. A train might travel 70 km per hour with respect to a stationary passenger, but as seen from the Sun the train's movement also includes the motion of the Earth as it orbits and spins. It's all relative.
- More often when talking about the movements of planets and spacecraft in the Solar System, we speak of their "orbital energy", a value which is directly related to the size of an object's orbit. Pluto, at the edge of the Solar System, travels in a much larger orbit than Mercury, the closest planet to the Sun, meaning Pluto's orbital energy is far, far greater than Mercury's.
- To get a spacecraft to Mercury, we therefore have to match its orbit. When BepiColombo was launched, its orbital energy was the same as our home planet's. Therefore, BepiColombo needs to either use a vast amount of propellant to ‘put on the brakes', or it can shed excess orbital energy by flying close to neighboring planets.
- The same works in reverse to voyage to the outer Solar System. To get into a larger orbit, further from the Sun, a spacecraft such as ESA's upcoming Juice mission to Jupiter will steal orbital energy from Earth, Venus and Mars.
It All Depends on your Frame of Reference
- So what's the difference between stealing energy from a planet and donating to it? It all depends on the relative motion of the spacecraft and the planet.
- From the perspective of a planet being flown by, the spacecraft comes towards it and leaves with the same velocity, only its path has been deflected. (The spacecraft has also deflected the planet, but by such a miniscule amount as to be insignificant. Nonetheless, Newton's third law of motion has been preserved: "To every action there is an equal and opposite reaction".)
- But remember, the planet itself is in motion around the Sun, and orbiting with a huge amount of momentum. As seen from the perspective of the Sun, the spacecraft is not just diverted on its path but it will have picked up or dropped off orbital energy depending on the geometry of the encounter.
- To increase speed with respect to the Sun, the spacecraft flies with the movement of the planet, acquiring some of the planet's orbital energy in the process; to decrease speed with respect to the Sun, the spacecraft flies against the movement of the planet to transfer some of its own orbital energy to the planet.
Orbital Mechanics: a Merging of Nature and Science
- To voyage through the Solar System requires more than simply a rocket, thrusters and propellant. Our teams of flight dynamics experts and flight control teams at ESA's Operation's Centre have to understand, work with, and get the best out of the motions of the planets and the unavoidable laws of nature.
- Find out about the double gravity assist of Venus taking place over 9-10 August, in which BepiColombo and Solar Orbiter both used the planet to alter their orbits, bringing them closer to Mercury and the Sun.
• August 2, 2021: Solar Orbiter and BepiColombo are set to make space history with two Venus flybys just 33 hours apart on 9 and 10 August. 49)
- The two spacecraft need the gravitational swingby to help them lose a little orbital energy in order to reach their destinations towards the centre of the Solar System. The double flyby also offers an unprecedented opportunity to study the Venus environment from different locations at same time and, moreover, in locations that are not typically visited by a dedicated planetary orbiter.
- Solar Orbiter, a partnership between ESA and NASA, will fly by Venus on 9 August with a closest approach of 7995 km at 04:42 UTC. Throughout its mission it makes repeated gravity assist flybys of Venus to get closer to the Sun, and to change its orbital inclination, boosting it out of the ecliptic plane, to get the best – and first – views of the Sun's poles.
- BepiColombo, a partnership between ESA and JAXA, will fly by Venus at 13:48 UTC on 10 August at an altitude of just 550 km. BepiColombo is on its way to the mysterious innermost planet of the solar system, Mercury. It needs flybys of Earth, Venus and Mercury itself, together with the spacecraft's solar electric propulsion system, to help steer into Mercury orbit against the immense gravitational pull of the Sun.
Photos or it Didn't Happen
- It is not possible to take high-resolution imagery of Venus with the science cameras onboard either mission – Solar Orbiter must remain facing the Sun, and the main camera onboard BepiColombo is shielded by the transfer module that will deliver the two planetary orbiters to Mercury. However, two of BepiColombo's three monitoring cameras will be taking photos around the time of close approach and in the days after as the planet fades from view.
- The cameras provide black-and-white snapshots in 1024 x 1024 pixel resolution, and are positioned on the Mercury Transfer Module such that they also capture the spacecraft's solar arrays and antennas. During the closest approach Venus will fill the entire field of view, but as the spacecraft changes its orientation the planet will be seen passing behind the spacecraft structural elements.
- The images will be downloaded in batches, one by one, with the first image expected to be available in the evening of 10 August, and the majority on 11 August.
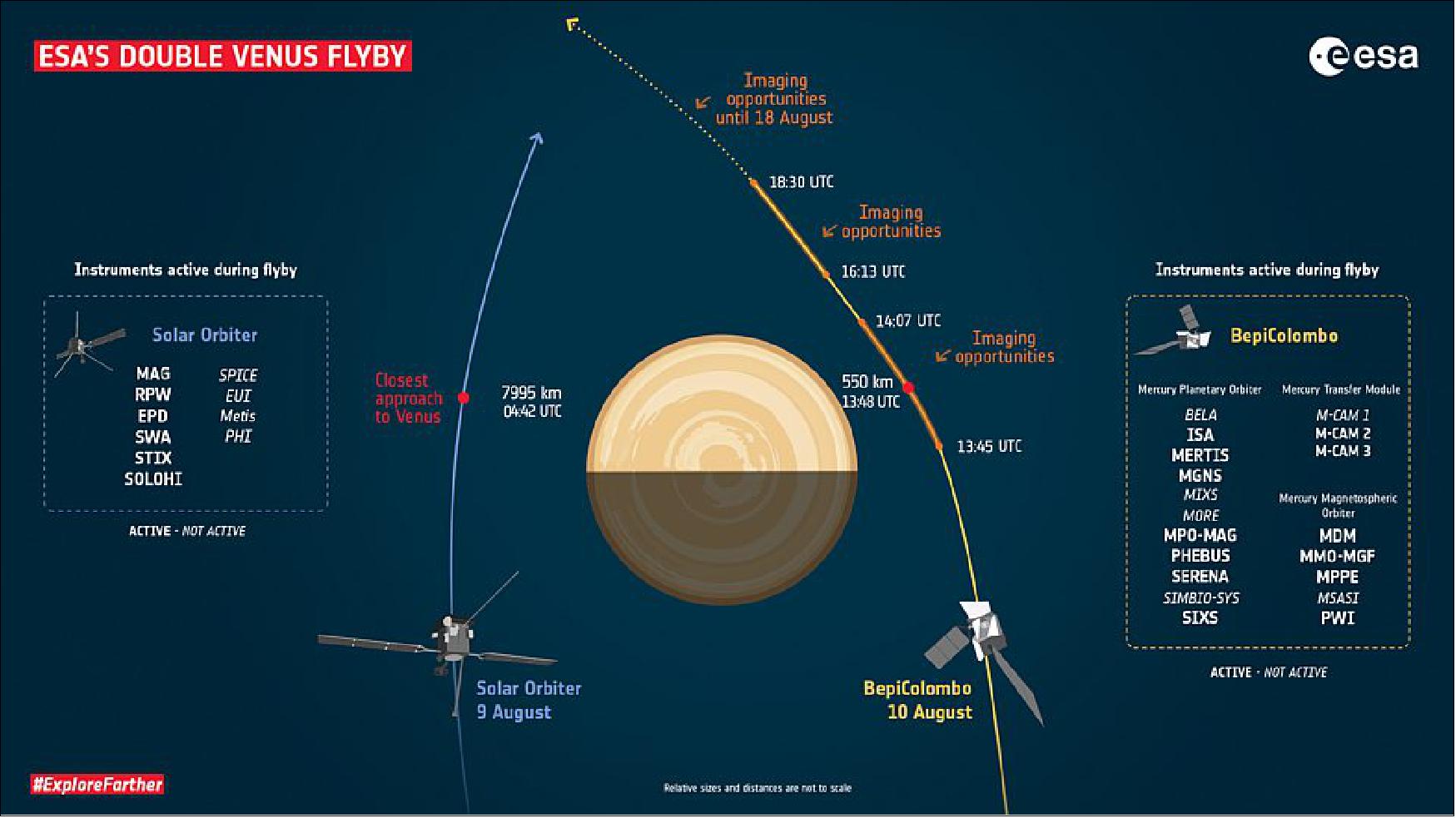
- Furthermore, there may be an opportunity for Solar Orbiter's SoloHI imager to observe the nightside of Venus in the week before closest approach. SoloHI usually takes images of the solar wind – the stream of charged particles constantly released from the Sun – by capturing the light scattered by electrons in the wind.
- It is – unfortunately! – not expected that one spacecraft will be able to image the other. Even at their closest the spacecraft will be more than 575 thousand km apart.
Multipoint Science
- Solar Orbiter has been acquiring data near-constantly since launch in February 2020 with its four in situ instruments that measure the environment around the spacecraft itself. Both Solar Orbiter and BepiColombo's Mercury Planetary Orbiter and Mercury Magnetospheric Orbiter will collect data on the magnetic and plasma environment of Venus from different locations. At the same time, JAXA's Akatsuki spacecraft is in orbit around Venus, creating a unique constellation of datapoints. It will take many months to collate the coordinated flyby measurements and analyse them in a meaningful way.
- The data collected during the flybys will also provide useful inputs to ESA's future Venus orbiter, EnVision, which was selected earlier this year and will launch to Venus in the 2030s.
Where to Next?
- Solar Orbiter and BepiColombo both have one more flyby this year.
- During the night of 1-2 October BepiColombo will see its destination for the first time, making its first of six flybys of Mercury – with this one from just 200 km distance. The two planetary orbiters will be delivered into Mercury orbit in late 2025, tasked with studying all aspects of this mysterious inner planet from its core to surface processes, magnetic field, and exosphere, to better understand the origin and evolution of a planet close to its parent star.
- On 27 November, Solar Orbiter will make a final flyby of Earth at 460 km, kicking off the start of its main mission. It will continue to make regular flybys of Venus to progressively increase its orbit inclination to best observe the Sun's uncharted polar regions, which is key to understanding the Sun's 11 year activity cycle.
• May 17, 2021: Combining imagery from three of Solar Orbiter's remote sensing instruments – the Extreme Ultraviolet Imager (EUI), the METIS coronagraph, and Solar Orbiter's Heliospheric Imager (SoloHI) – provides both close-up and wide views of the evolution of a coronal mass ejection (CME) on 12-13 February 2021. CMEs are eruptions of particles from the solar atmosphere that blast out into the Solar System. 50)
- Solar Orbiter is a space mission of international collaboration between ESA and NASA.
• April 27, 2021: Computer simulations show that the miniature solar flares nicknamed ‘campfires', discovered last year by ESA's Solar Orbiter, are likely driven by a process that may contribute significantly to the heating of the Sun's outer atmosphere, or corona. If confirmed by further observations this adds a key piece to the puzzle of what heats the solar corona – one of the biggest mysteries in solar physics. 51)
Mystery Heating
- The Sun has a mysterious feature: somehow the tenuous outer atmosphere contains gas with a temperature of a million degrees, yet the solar surface is just 5500°C. Logic would suggest that if you have a body that is very hot at the center and relatively cool on the surface, it should be even cooler the further you go away. But the peculiar thing about the corona of the Sun – and many other stars as well – is that it starts to heat up the further you move above the surface. Many ideas have been put forward over the last decades homing in on the Sun's magnetic field, but how the energy is generated, transported and dissipated has been a source of much debate.
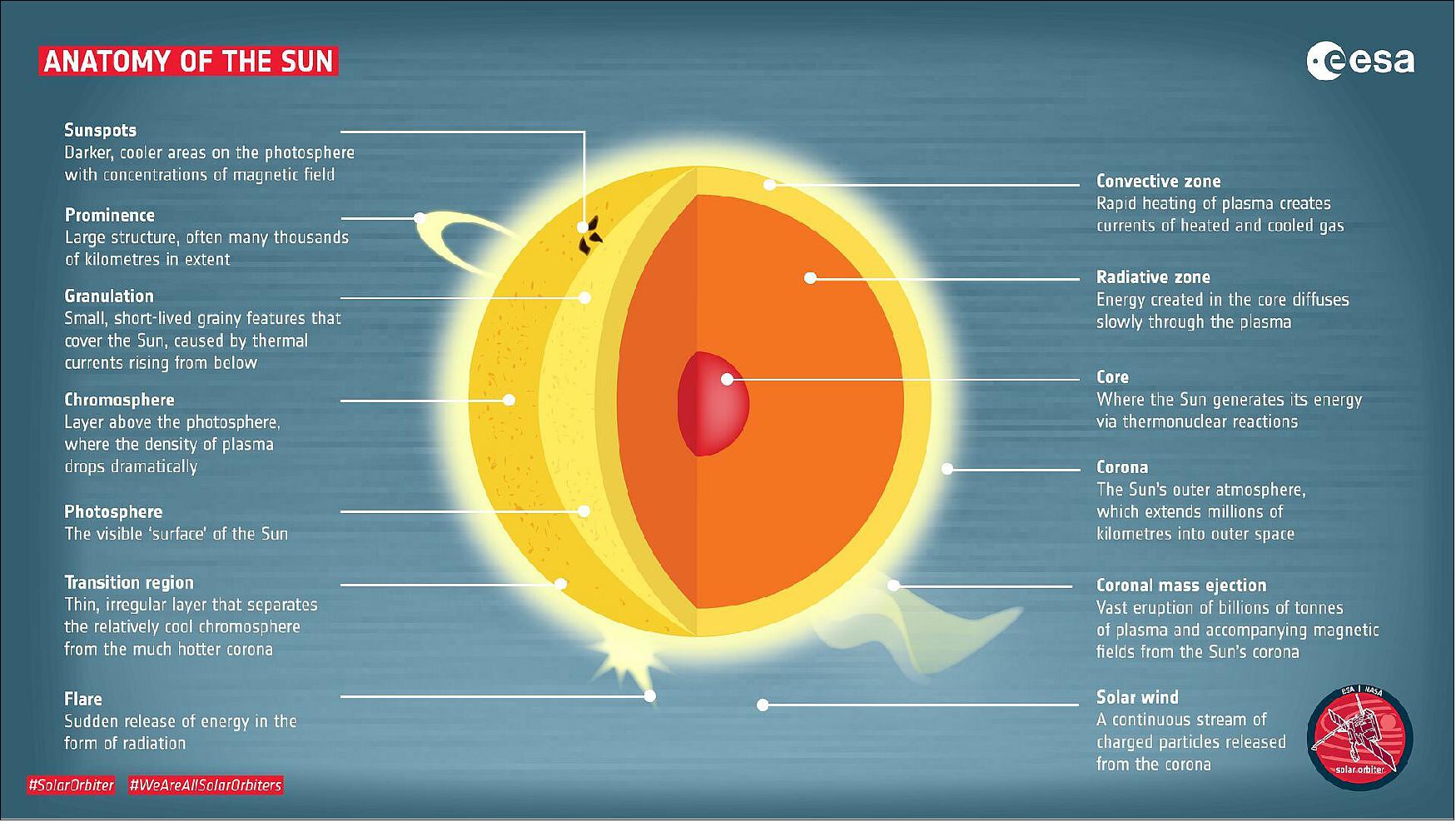
• Core: This is where the Sun generates its energy. The temperature in the core is around 15 million degrees Celsius. This, combined with the huge pressure and density of the plasma force hydrogen nuclei to fuse together, creating helium and releasing vast quantities of energy in the process. Every second, the Sun converts four million tonnes of matter into energy in this way, which begins a slow journey towards the surface. • Radiative zone: This is the layer above the core. Although not as dense as the core, the plasma is still packed so tightly in the radiative zone that convection cannot take place. Instead, the energy created in the core diffuses slowly through the plasma. It takes photons around 170,000 years to pass through the radiative zone: The photons travel at the speed of light, but can travel only a few millimeters at a time before they are absorbed by an atom and then re-emitted in any direction. At the top of the zone, the temperature is around two million degrees Celsius. At the base, next to the Sun's core, the temperature is around seven million degrees Celsius. • Convective zone: This lies between the deeper, radiative zone and the photosphere. The convective zone is 200,000 km deep. While the top layer is the same temperature as the photosphere (between 4500 – 6000 degrees Celsius), the base of the convective zone reaches two million degrees Celsius. Plasma at the base of the zone is heated rapidly. This makes it buoyant and so it rises rapidly, creating a turbulent convection pattern, rather like a boiling pan of water – only 200,000 km deep and surrounding the entire Sun. • Photosphere: This is the visible ‘surface' of the Sun. Almost all radiation from the Sun is emitted from this thin layer of several 100 km thickness, which lies at the upper boundary of the convection zone. It is where the energy generated in the core can finally move freely through space. The temperature of the photosphere varies from place to place but lies between 4500 and 6000 degrees Celsius. • Chromosphere: This is the layer above the photosphere, where the density of plasma drops dramatically. In general, the chromosphere is roughly 1000–2000 kilometers thick, with a temperature that rises from around 4000 to about 25 000 degrees Celsius. Spires of chromospheric gas, known as spicules, can reach up to a height of 10,000 km. • Transition region: This is a thin, irregular layer that separates the relatively cool chromosphere from the much hotter corona. Across the transition zone, the temperature of the solar plasma soars to nearly a million degrees Celsius. While the convection zone and partly also the solar photosphere are dominated by flows which are capable of moving regions of strong magnetic flux around, the transition region and corona are dominated by the magnetic field which forces the plasma to move predominantly along field lines. • Corona: This is the Sun's outer atmosphere and extends to millions of kilometers into outer space. It is most easily seen during a total solar eclipse. The plasma in the corona is extremely hot at more than one million degrees Celsius, yet is very rarefied. Its density is typically just one trillionth of the density of the photosphere. The solar wind originates in the corona. • Prominence: These are large structures, often thousands of kilometers in extent. They are made of tangled magnetic field lines that keep dense concentrations of solar plasma suspended above the Sun's surface and often take the form of loops that arch up from the chromosphere. They can persist for several weeks or even months. • Solar flare: This is a sudden release of energy. A flare is usually created when the magnetic field lines that make sunspots transform themselves rapidly into more stable configurations. This is a bit like a stretched elastic band breaking and releasing all of its stored energy as it snaps back into position. The energy released by solar flares strongly influences the behavior of the solar wind. • Sunspots: These are temporary features on the photosphere. They look like dark patches against the brighter region of the photosphere because they about 1000 degrees cooler and so do not emit so much light. They are caused by magnetic fields breaking through the photosphere of the Sun and cooling the gas there. Sunspots can be anything from a few tens of kilometers across to larger than 150,000 km. • Granulation: These are convective patterns that occur in the photosphere. Each granule is about 1000 km wide and consists of hot plasma rising in its centre. As it releases its energy into space, the plasma cools and this makes it flow to the sides of the granule and sink back down into the photosphere. Individual granules persist for about 20 minutes, after this new ones in slightly different places develop. • Coronal mass ejections: These are vast eruptions of billions of tonnes of plasma and magnetic fields from the Sun's corona. They travel out from the Sun at speeds of hundreds to thousands of kilometers per second, and if sent into the pathway of the Earth, can create geomagnetic storms. |
Zooming in on Solar Campfires
- The movie begins with a spectacular view of the Sun as seen by EUI's Full Sun Imager. It shows the transition region of the Sun, at nearly 100 000ºC, which separates the relatively cool chromosphere (the layer above the photosphere, the visible surface of the Sun) from the much hotter corona (the Sun's outer atmosphere, which extends millions of kilometers into space).
- The view then fades to show the low corona of the Sun, which is heated to about 1 million degrees. Zooming in with the High Resolution Imager in extreme ultraviolet light (yellow colors) highlights details of the corona. A sliding side-by-side comparison with the cool solar chromosphere follows, imaged by the Lyman alpha channel of the High Resolution Imager (pink colors).
- Finally, zooming in at extreme ultraviolet wavelengths reveals solar campfires, which are the smallest, bright loops in these images. They are likened to miniature solar flares, manifesting as short-lived brightenings in the lower corona, and appear to be rooted in the magnetic flux concentrations of the chromosphere.
- Computer simulations replicating the campfires suggests that they may play a role in heating the corona.
- Stunning detail already provided by Solar Orbiter's Extreme Ultraviolet Imager (EUI) ‘first light' images just months after launch last year and since then has revealed more than 1500 small, flickering brightenings nicknamed campfires. These short-lived campfires last for between 10 and 200 seconds, and have a footprint covering between 400 and 4000 km. The smallest and weakest events, which had not been observed before, seem to be the most abundant, and represent a previously unseen fine structure of the region where the heating mystery is suspected to be rooted.
Model Campfires
- Yajie Chen, a PhD student from Peking University in China, working with Professor Hardi Peter from the Max Planck Institute for Solar System Research in Germany and colleagues, used a computer model to dive into the physics of the campfires, with exciting first results.
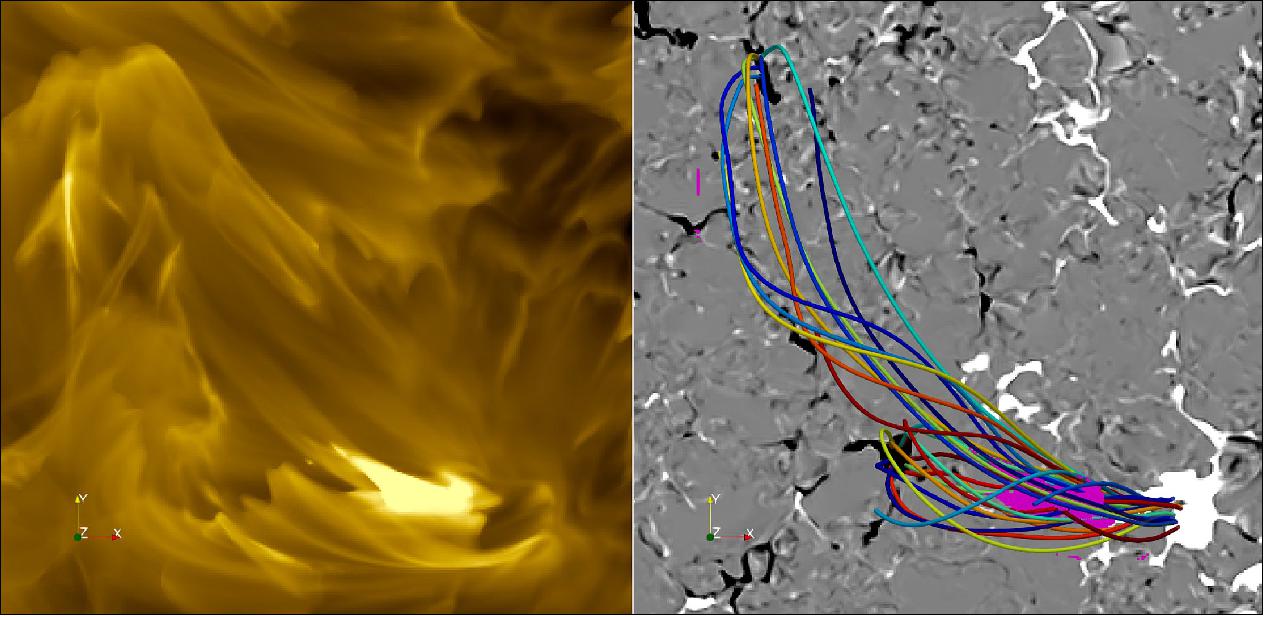
- In both images (Figure 53 and 54) the left panel shows a simulated view of emission from the Sun's corona, as would be seen by Solar Orbiter's Extreme Ultraviolet Imager. The right panel shows the corresponding magnetic field as a magnetogram, where black and white are opposite polarities, and grey is zero field. Overplotted on the magnetogram are selected field lines in different colors.
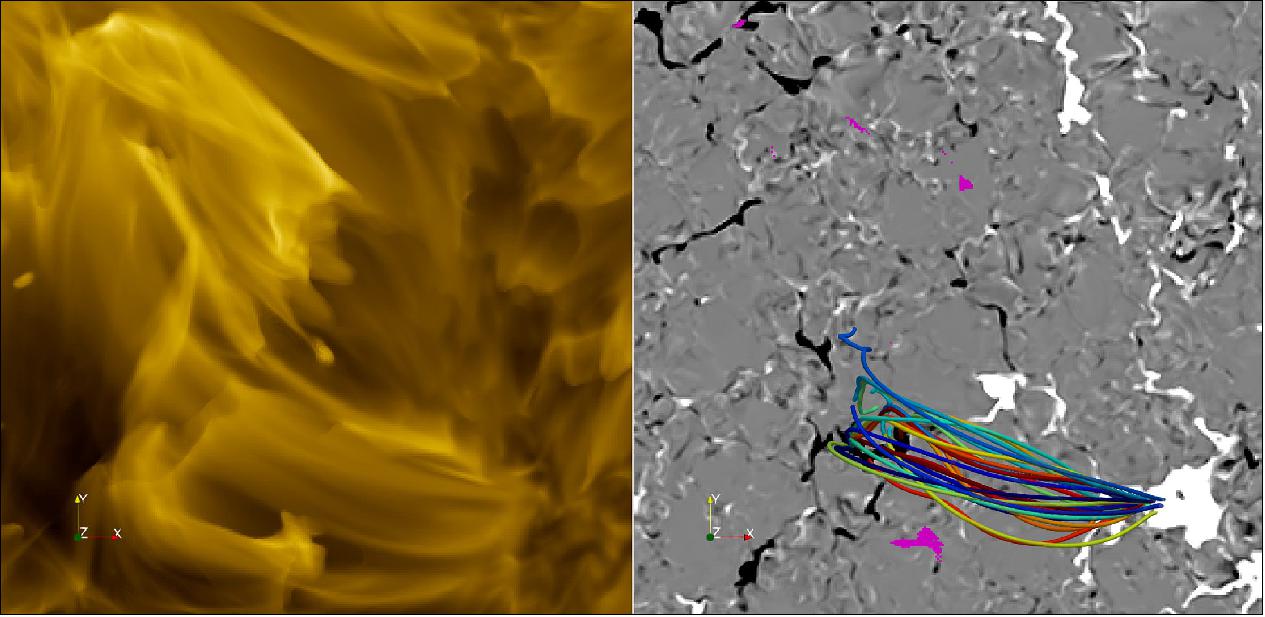
- "Our model calculates the emission, or energy, from the Sun as you would expect a real instrument to measure," explains Hardi. "The model generated brightenings just like the campfires. Furthermore, it traces out the magnetic field lines, allowing us to see the changes of the magnetic field in and around the brightening events over time, telling us that a process called component reconnection seems to be at work."
- Reconnection is a well-known phenomenon whereby magnetic field lines of opposite direction break and then reconnect, releasing energy when they do so. Typical reconnection happens between field lines pointing in opposite directions, but with so-called component reconnection the field lines are almost parallel, pointing in a similar direction, with reconnection therefore happening at very small angles.
- "In one of our case studies, we find that the untwisting of a flux rope [helical magnetic field lines winding around a common axis] initiates the heating instead," adds Hardi. "It's exciting to find these variations, and we're looking forward to see what further insights our models bring to help us improve our theories on the processes behind the heating."
- The team cautions that it's very early days. They have used the model to look at seven of the brightest events generated in their simulation, which likely correspond to the largest campfires observed by EUI. Key to advancing the study will be joint observations between EUI and the spacecraft's Polarimetric and Helioseismic Imager (PHI) and Spectral Imaging of the Coronal Environment (SPICE) spectrograph once Solar Orbiter's full science mission gets going in November. PHI will reveal the magnetic field of the Sun and how it changes on the surface, while SPICE will measure the temperature and density of the corona.
Teamwork
- Further insight into the campfires has also been enabled by pairing up with NASA's Solar Dynamics Observatory (SDO), which is in orbit around the Earth, to triangulate the height of the campfires in the solar atmosphere.
- "To our surprise, campfires are located very low in the solar atmosphere, only a few thousand kilometers above the solar surface, the photosphere," says David Berghmans, Principal Investigator of EUI. "It is very early days, and we are still learning a lot about the campfire characteristics. For example, even though campfires look like small coronal loops, their length is on average a bit short for their height, suggesting we only see part of these little loops. But our preliminary analysis also shows that campfires do not really change their height during their lifetime, setting them aside from jet-like features."
- Understanding the characteristics of the campfires and their place amongst other known solar phenomena will enable scientists to dive deeper into the solar corona heating problem.
![Figure 56: What do we know about solar campfires so far? This graphic provides a summary of what ESA's Solar Orbiter mission, as well as computer modelling, has revealed about solar campfires in the first year of the mission. Campfires are miniature solar flares manifesting as short-lived brightenings in the lower corona, rooted in the magnetic flux concentrations of the chromosphere. They were first identified in Extreme Ultraviolet Imager data, and computer simulations are providing insights into the magnetic field phenomena driving them [image credit: Sun image: Solar Orbiter/EUI Team/ESA & NASA; Data: Berghmans et al (2021) and Chen et al (2021)]](https://eoportal.org/ftp/satellite-missions/s/SolO_200522/SolO_Auto3F.jpeg)
- "How fantastic to already have such promising data that may provide insight into one of solar physics' greatest mysteries before Solar Orbiter has even begun its nominal science phase," says ESA's Solar Orbiter Project Scientist Daniel Müller. "Our mission is lucky to be building on the incredible ground-work of those that have flown before, and the theories and models already put forward over the last decades. We're looking forward to see what missing details Solar Orbiter – and the solar community working with our data – will contribute to solving open questions in this exciting field."
- Solar Orbiter is currently in ‘cruise phase', focused primarily on instrument calibration, and will begin coordinated observations between its suite of ten remote sensing and in situ instruments from November this year.
- Solar Orbiter is a space mission of international collaboration between ESA and NASA.
- 'Campfires' is one of many subjects being discussed in a dedicated Solar Orbiter first results session at the European Geosciences Union (EGU) General Assembly today (27 April 2021).
- The results were discussed at EGU today and are linked to the following publications: 52) 53)
• February 9, 2021: What happens when the Solar System's No. 1 source of violent energy interferes with spacecraft communication? 54)
- Name? Solar Orbiter, or ‘Solo' as the mission control team fondly call it, is one of the European Space Agency's pluckiest missions and is now cruising toward the Sun.
- Age: One year old! We launched on 10 February 2020. Granted, it was first powered up on Earth at some point during construction, but launch is ‘when it came alive'.
- What's it doing out there? It's imaging our star, observing the solar wind and unravelling mysteries of the solar cycle. It's already returned some of the best images of ol'Sol ever, revealing omnipresent miniature solar flares, dubbed ‘campfires', near the surface.
- Anything else? Well, it uses prehistoric cave pigment as a coating to withstand temperatures up to 520ºC. The Sun's pretty darn hot, you know.
- So, what's happening now? The spacecraft's orbit is taking it behind the Sun, and starting a few days ago the apparent angle, as seen from Earth, between Solar Orbiter and the Sun started falling below 5 degrees. It's called ‘conjunction season' and runs until mid-February.
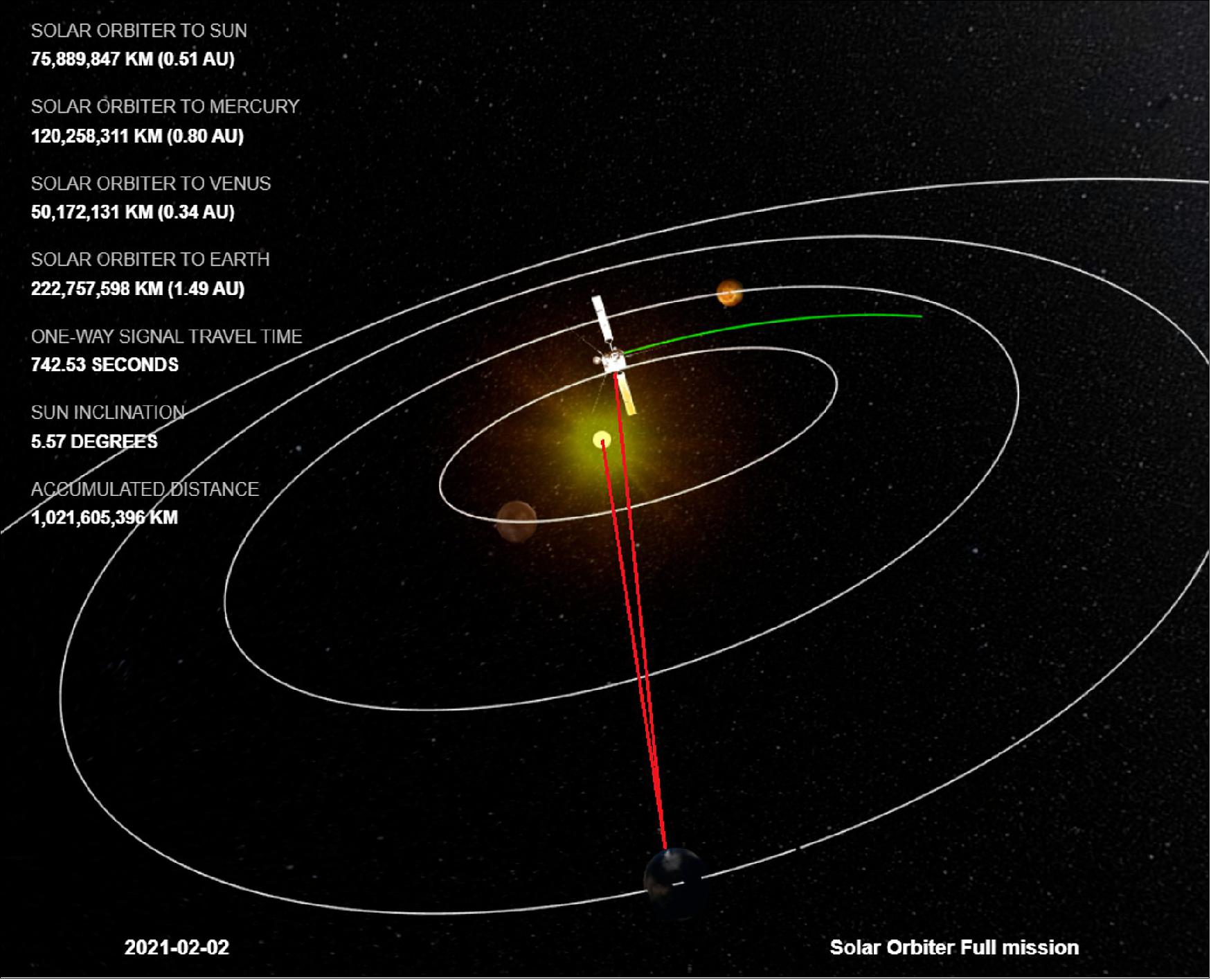
- Ohhh, will it melt? No. See the bit about cave pigment above. But the Sun's energetic and unpredictable atmosphere, aka heliosphere, will start making it hard for radio dish antennas on Earth – which will also have to point to the Sun when pointing at Solar Orbiter – to reliably send or receive signals, or do so very fast, at any rate.
- Sounds bad. It is – the Sun's energy can disrupt communication in both directions. Mission controllers will only be able to receive data at about 255 bits per second, or send signals at about 7.8 bits per second. You're likely too young to remember dial-up modems.
- Not fast enough for Netflix, but it's something, isn't it? Perhaps, but even these piddly data rates aren't reliable – the radio link might be lost entirely if the Sun so much as even sneezes.
- If the bit rate is so low due to interference from the Sun, why not simply turn off the instruments and coast through the worst part of conjunction? That's what happens with other, older missions using simpler technology, not to mention Mars Express. And not that there's any friendly competition between mission control teams.
- OK, what's the good news? Our mission planners knew this would happen, so Solar Orbiter was designed to endure long periods of no contact with Earth. The spacecraft can even keep its scientific instruments running autonomously, and simply store all the collected data on board for download later, away from the Sun.
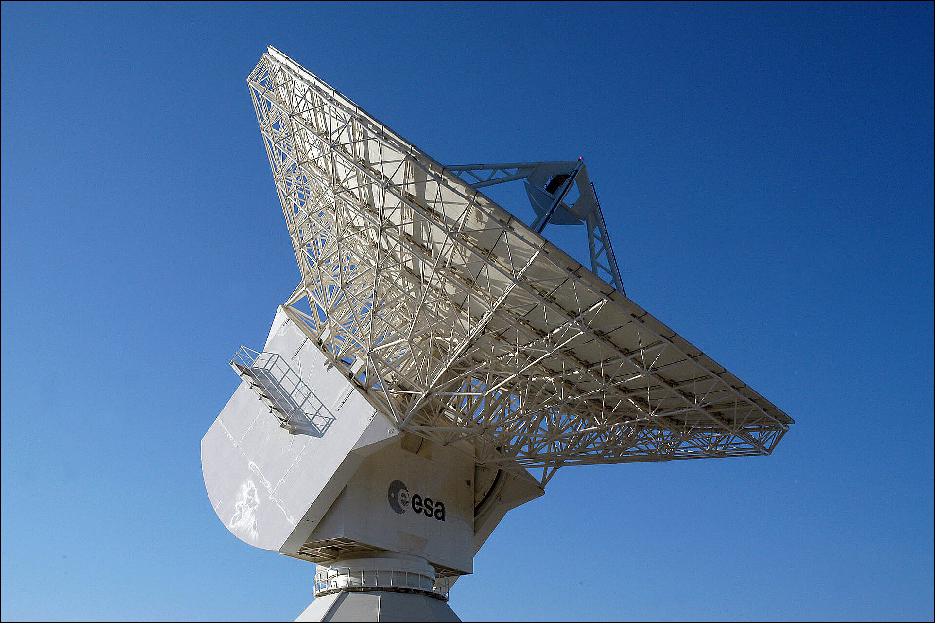
- I bet there was a rush to get ready for this. We're spacecraft engineers; we never panic, but we are very good at dealing with contingencies. Which this is not. But, yes, many of us at ESA worked with a ‘sense of urgency' to prepare and upload three week's worth of onboard instructions, in case we had no contact at all during conjunction. All while dealing with the critical Venus flyby maneuver in December, while uploading a complete new operating system and all while working from our kitchen tables.
- Now that your bird's on autopilot, there's not much to do? Au contraire. We've got ‘remote science checkout during solar close approach' coming up, as well as a special ‘thermal characterization' campaign requiring ‘new telemetry modes' followed by more testing of the radio communication link. Then there are the Venus and Earth flybys later this year, after which we have to get ready for the final science orbit at the end of cruise. Phew!
- What's most often heard in the team's Skype chats? "Cruise was supposed to be quiet ..." followed by "... please mute your mic – we hear the cat meowing under the kitchen table...!"
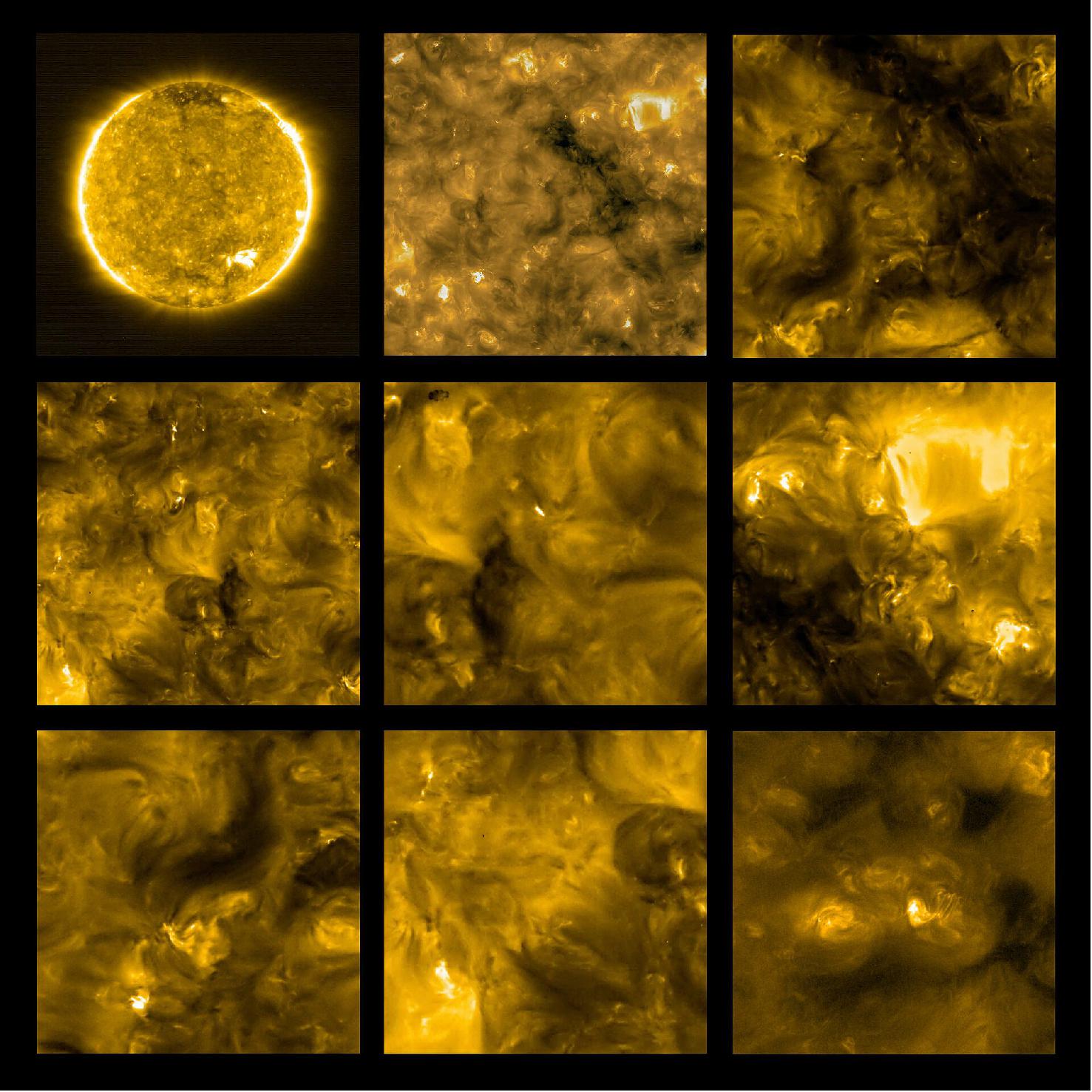
• January 26, 2021: Three Solar System planets are visible in the foreground of Figure 60: Venus (left), Earth (middle), and Mars (right). Stars are visible in the background. 55)
- Venus is the brightest object in the video, roughly 48 million km away from Solar Orbiter. The distance to Earth was 251 million km and 332 million km to Mars on that day. The Sun is located on the right, outside the video frame.
- At the moment of the recording, Solar Orbiter was on its way to Venus for its first gravity assist flyby, which happened on 27 December. Venus and Earth flybys will bring the spacecraft closer to the Sun and tilt its orbit in order to observe our star from different perspectives.
- Solar Orbiter is the most complex scientific laboratory ever to have been built to study the Sun and the solar wind, taking images of our star from closer than any spacecraft before. During its initial cruise phase, which lasts until November 2021, Solar Orbiter is already acquiring data constantly with its four in situ instruments. These instruments measure the conditions around the spacecraft itself.
- SoloHI is one of the six remote-sensing instruments onboard the mission. During cruise phase, these are still being calibrated during specific periods, but are switched off otherwise. SoloHI takes images of the solar wind – the stream of charged particles constantly released by the Sun into outer space – by capturing the light scattered by electrons in the wind.
- The solar wind, along with powerful ejections of plasma from the Sun, can cause disturbances in our space environment – a phenomenon called "space weather" – which can potentially harm astronauts, satellites in space and disrupt ground-based technology.
- Understanding what drives the solar wind and the acceleration of solar wind particles, will helps us better predict periods of stormy space weather.
- Where is Solar Orbiter now? Track the location of Solar Orbiter from home using this interactive chart!
- Solar Orbiter is a space mission of international collaboration between ESA and NASA.
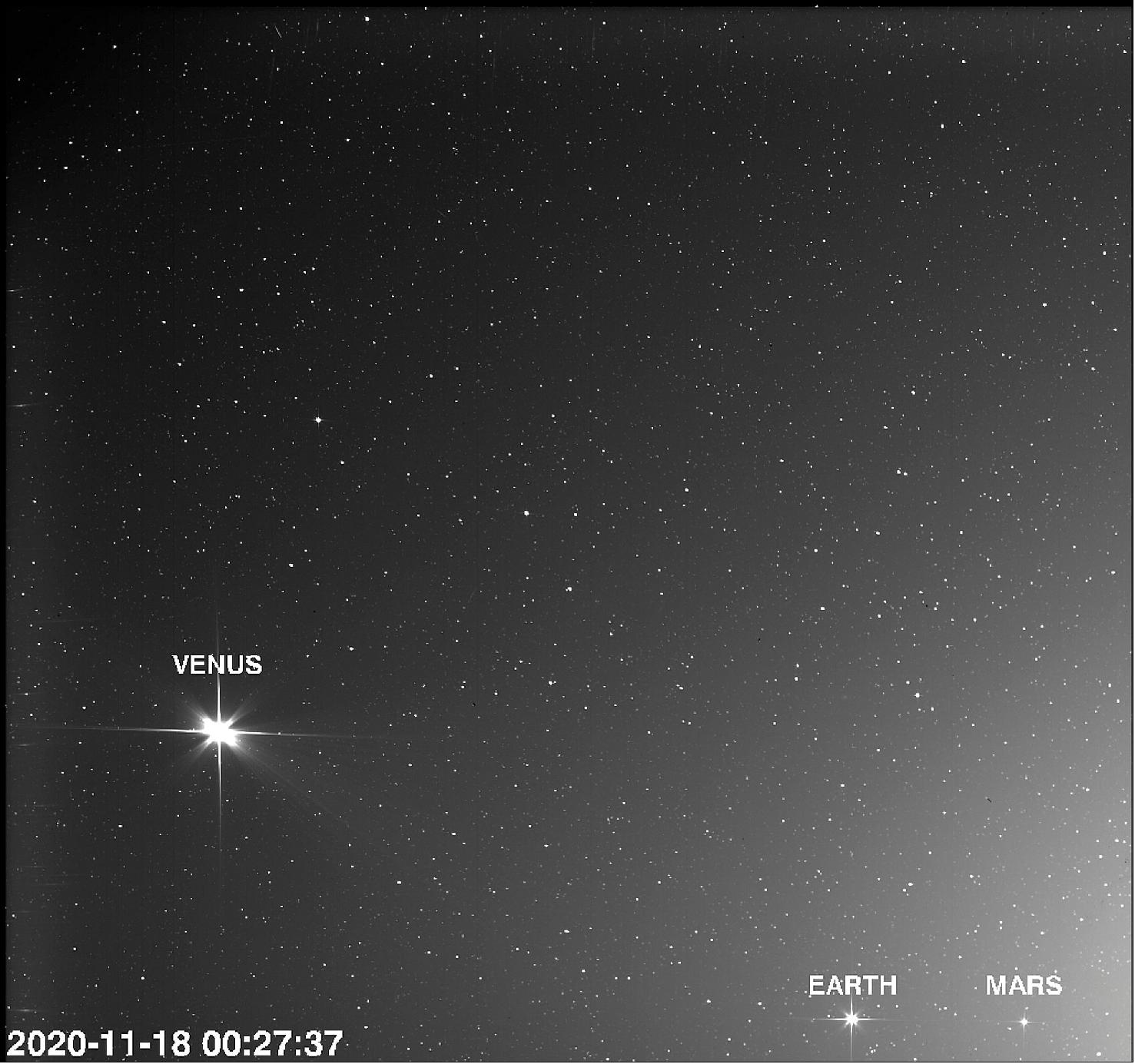
• December 17, 2020: Solar Orbiter is getting ready for the first of many gravity assist flybys of Venus on 27 December, to start bringing it closer to the Sun and tilting its orbit in order to observe our star from different perspectives. 56)
- Just as the majority of us will remain safely at home under various COVID-19 pandemic lockdown measures during what is traditionally a holiday period, the flyby – a routine event in the world of flying spacecraft – will also be monitored by the spacecraft operations managers remotely as well.
- Closest approach will take place at 12:39 UTC (13:39 CET) on 27 December, and will see the spacecraft fly some 7 500 km from the Venus cloud tops. Later flybys, from 2025, will see much closer encounters of just a few hundred kilometers (see Figure 66).
- During the upcoming flyby several in-situ science instruments – MAG, RPW and some sensors of EPD – will be switched on to record the magnetic, plasma and particle environment around the spacecraft as it encounters Venus. (It is not possible to take images of Venus during the flyby because the spacecraft must remain facing the Sun.)
- In order to properly line up for the flyby, specialists from ESA's ground stations and flight dynamics teams conducted a so-called ‘Delta-DOR' campaign, using an advanced technique – Delta-Differential One-Way Ranging – to precisely determine the spacecraft's position in space, and its trajectory.
- In Delta-DOR, a set of widely separated ground stations on Earth are used to receive the spacecraft's radio signals, giving a first result for its location. Then, this result is compared to locations of known stellar radio sources previously mapped by other missions, resulting in a corrected and ultra-precise final plot. The Delta-DOR technique allows operators to determine where a spacecraft is to within a few hundred meters, even at a distance of 100 million km.
- Today, 17 December, Solar Orbiter is 235 million kilometers from Earth, and about 10.5 million from Venus. It takes about 13 minutes for signals to travel to (or from) the spacecraft.
- Solar Orbiter's path around the Sun has been chosen to be ‘in resonance' with Venus, which means that it will return to the planet's vicinity every few orbits and can again use the planet's gravity to alter or tilt its orbit. The next encounter will be in August 2021, which is also within a few days of BepiColombo's next Venus gravity assist. Initially Solar Orbiter will be confined to the same plane as the planets, but each encounter of Venus will increase its orbital inclination. By 2025 it will make its first solar pass at 17º inclination, increasing to 33º by the end of the decade, bringing even more of the polar regions into direct view. This will result in the spacecraft being able to take the first ever images of the Sun's polar regions, crucial for understanding how the Sun ‘works', for investigating the Sun-Earth connection and how we can better predict periods of stormy space weather.
- Solar Orbiter is a space mission of international collaboration between ESA and NASA.
• December 10, 2020: Solar Orbiter's latest results show that the mission is making the first direct connections between events at the solar surface and what's happening in interplanetary space around the spacecraft. It is also giving us new insights into solar ‘campfires', space weather and disintegrating comets. 57)
- "I could not be more pleased with the performance of Solar Orbiter and the various teams that keep it and its instruments operating," says Daniel Müller, ESA Solar Orbiter Project Scientist.
- "It has been a real team effort under difficult circumstances this year, and now we are beginning to see those efforts really paying off."
- Solar Orbiter's ten scientific instruments are split into two groups. There are six remote sensing telescopes, and four in-situ instruments. The remote sensing instruments look at the Sun and its extended atmosphere, the corona. The in-situ instruments measure the particles around the spacecraft, which have been released by the Sun and are known as the solar wind, along with its magnetic and electric fields. Tracing the origin of those particles and fields back to the solar surface is one of the key objectives of Solar Orbiter.
- During Solar Orbiter's first close pass of the Sun, which took place on 15 June and saw the spacecraft approach to 77 million kilometers, both remote sensing and in-situ instruments were recording data.
Footprints of the Solar Wind
- Solar Orbiter data have made it possible to calculate the source region of the solar wind that hits the spacecraft, and identify this ‘footprint' in the remote sensing images. In an example studied in June 2020, the footprint is seen at the edge of a region called a ‘coronal hole', where the Sun's magnetic field reaches out into space, allowing the solar wind to flow.
- Even though the work is preliminary, it is still beyond anything that has been possible so far.
- "We've never been able to do mapping this accurate before," says Tim Horbury, Imperial College, London, and Chair of the Solar Orbiter In-Situ Working Group.
Campfire Physics
- Solar Orbiter also has new information about the Sun's ‘campfires' that captured the world's attention earlier this year.
- The mission's first images showed a multitude of what appeared to be tiny solar eruptions bursting across the surface of the Sun. The scientists called them campfires because the exact energy associated with these events is not yet known. Without the energy, it is not yet clear whether they are the same phenomenon as other smaller-scale eruptive events that have been seen by other missions. What makes it all so tantalizing is that small-scale ‘nano-flares' have long been thought to exist on the Sun but we've never had the means to see events this small before.
- "The campfires could be the nano-flares that we are after with Solar Orbiter," says Frédéric Auchère, Institut d'Astrophysique Spatiale, Orsay, France, and Chair of the Solar Orbiter Remote-Sensing Working Group.
- This is important because the nano-flares are theorized to be responsible for heating the corona, the outer atmosphere of the Sun. The fact that the corona is at about a million degrees Celsius whereas the surface is only about 5000 degrees is still one of the most puzzling issues in solar physics today. Investigating this mystery is one of the key scientific objectives of Solar Orbiter.
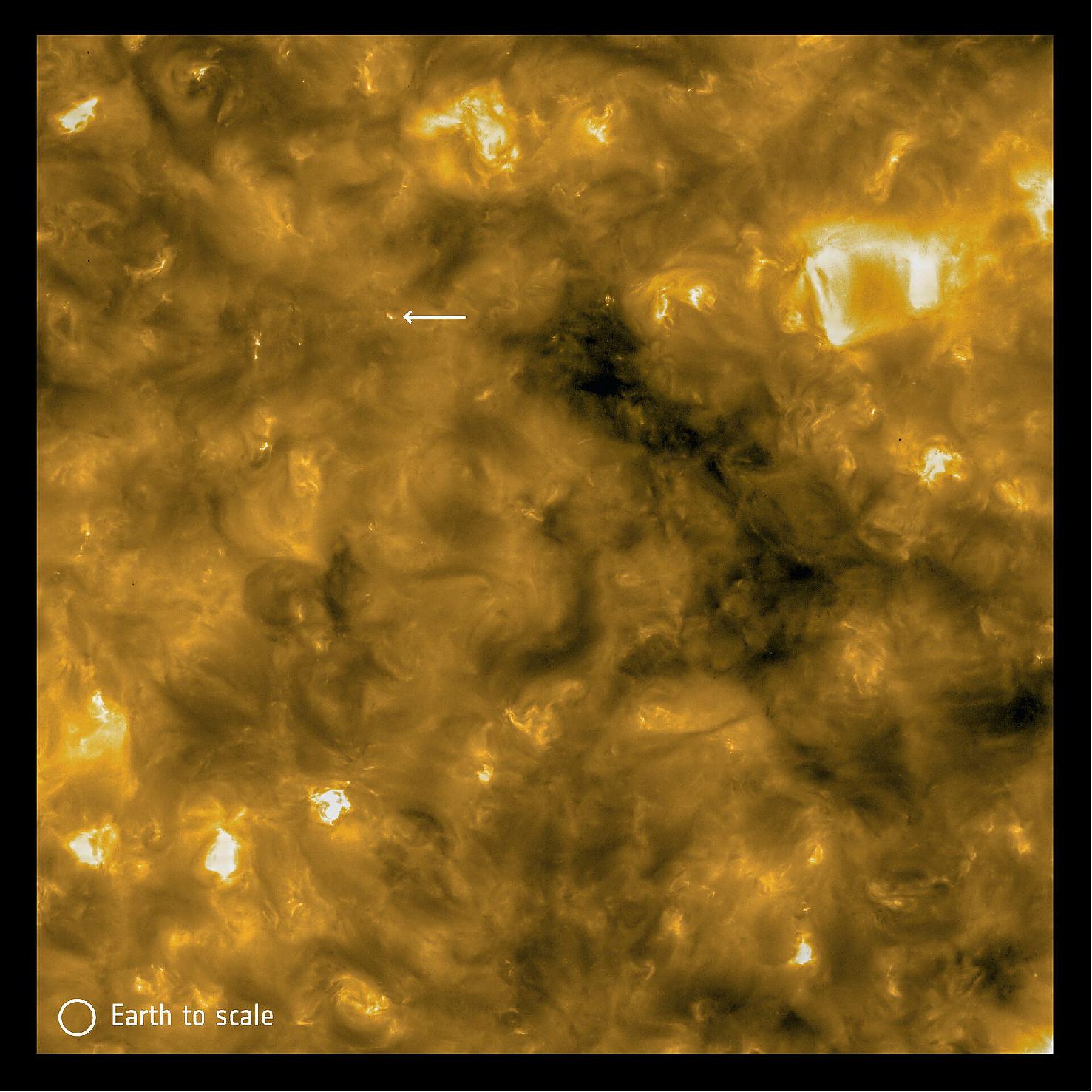
- To explore the idea, researchers have been analyzing data by Solar Orbiter's SPICE (Spectral Imaging of the Coronal Environment) instrument. SPICE is designed to reveal the velocity of the gas at the solar surface. It has shown that there are indeed small-scale events in which the gas is moving with significant velocity but looking for a correlation to the campfires has not yet been done.
- "Right now, we only have commissioning data, taken when the teams were still learning the behaviors of their instruments in space, and the results are very preliminary. But clearly, we do see very interesting things," says Frédéric. "Solar Orbiter is all about discovery, and that is very exciting."
Surfing a Comet's Tail
- As well as progress towards the planned scientific objectives of Solar Orbiter, there has also been serendipitous science from the spacecraft too.
- Shortly after Solar Orbiter was launched, it was noticed that it would fly downstream of Comet ATLAS, passing through its two tails. Although Solar Orbiter was not designed for such an encounter, and was not due to be taking science data at this time, mission experts worked to ensure that all the in-situ instruments did record the unique encounter.
- But Nature had one more trick to play: the comet disintegrated before the spacecraft got close. So, instead of the hoped-for strong signals from the tails, it was entirely possible that the spacecraft would see nothing at all.
- That was not the case. Solar Orbiter did see signatures in the data from comet ATLAS, but not the kind of things that scientists would normally expect. Instead of a strong, single tail-crossing, the spacecraft detected numerous episodes of waves in the magnetic data. It also detected dust in patches too. This was probably released from the insides of the comet as it split into many small pieces.
- "This is the first time we've essentially traveled through the wake of a comet that's disintegrated," says Tim. "There's a lot of really interesting data there, and it's another example of the kind of high-quality fortuitous science we can do with Solar Orbiter."
![Figure 64: An orbit's worth of particle data. Solar Orbiter's Energetic Particle Detector (EPD) has been switched on and collecting data since March 2020. It has now collected an entire orbit's worth of data. EPD measures the energetic particles that zip past the spacecraft. It looks at their composition and variation in time. The data will help scientists investigate the sources, acceleration mechanisms, and transport processes of these particles. - The diagram shows the flux of particles captured by the spacecraft during its first orbit (the earliest data is not included to avoid overlap on this diagram). The flux is calculated from the rate at which energetic particles are entering the instrument and represents space weather events in the solar wind. The spikes indicate the fluxes of electrons (shown as spikes pointing inwards towards the Sun in this representation) and ions (spikes pointing outwards), on a logarithmic scale. - The solar wind is the constant stream of particles that flows away from the Sun. Energetic events such as solar flares and coronal mass ejections can accelerate and release large quantities of high-energy particles. Although the Sun has been relatively inactive this year, there have still been a number of space weather events that had dramatically increased the number of energetic particles flowing past Solar Orbiter. - Although this plot comes from EPD, the three other in-situ instruments on Solar Orbiter are also designed to study these events in unprecedented detail. The three other instruments are Magnetometer (MAG), Radio and Plasma Waves (RPW), and Solar Wind Plasma Analyzer (SWA). - By taking these state-of-the-art instruments close to the Sun, Solar Orbiter is able to use high data rates to capture the details with more fidelity than ever before. In the case of the EPD, researchers are already seeing rapid variations in the number of particles that are associated with changes in the magnetic field, as seen by MAG. This is revealing fine scale structures and new physical interactions in the solar wind that we have never seen before [image credit: Solar Orbiter/EPD (ESA & NASA)]](https://eoportal.org/ftp/satellite-missions/s/SolO_200522/SolO_Auto39.jpeg)
Stealth Space Weather
- Solar Orbiter has been measuring the solar wind for much of its time in space, recording a number of particle ejections from the Sun. Then, on 19 April, a particularly interesting coronal mass ejection swept across Solar Orbiter.
- A coronal mass ejection, or CME, is a large space weather event, in which billions of tonnes of particles can be ejected from the Sun's outer atmosphere. During this particular CME, which burst from the Sun on 14 April, Solar Orbiter was about twenty percent of the way from the Earth to the Sun.
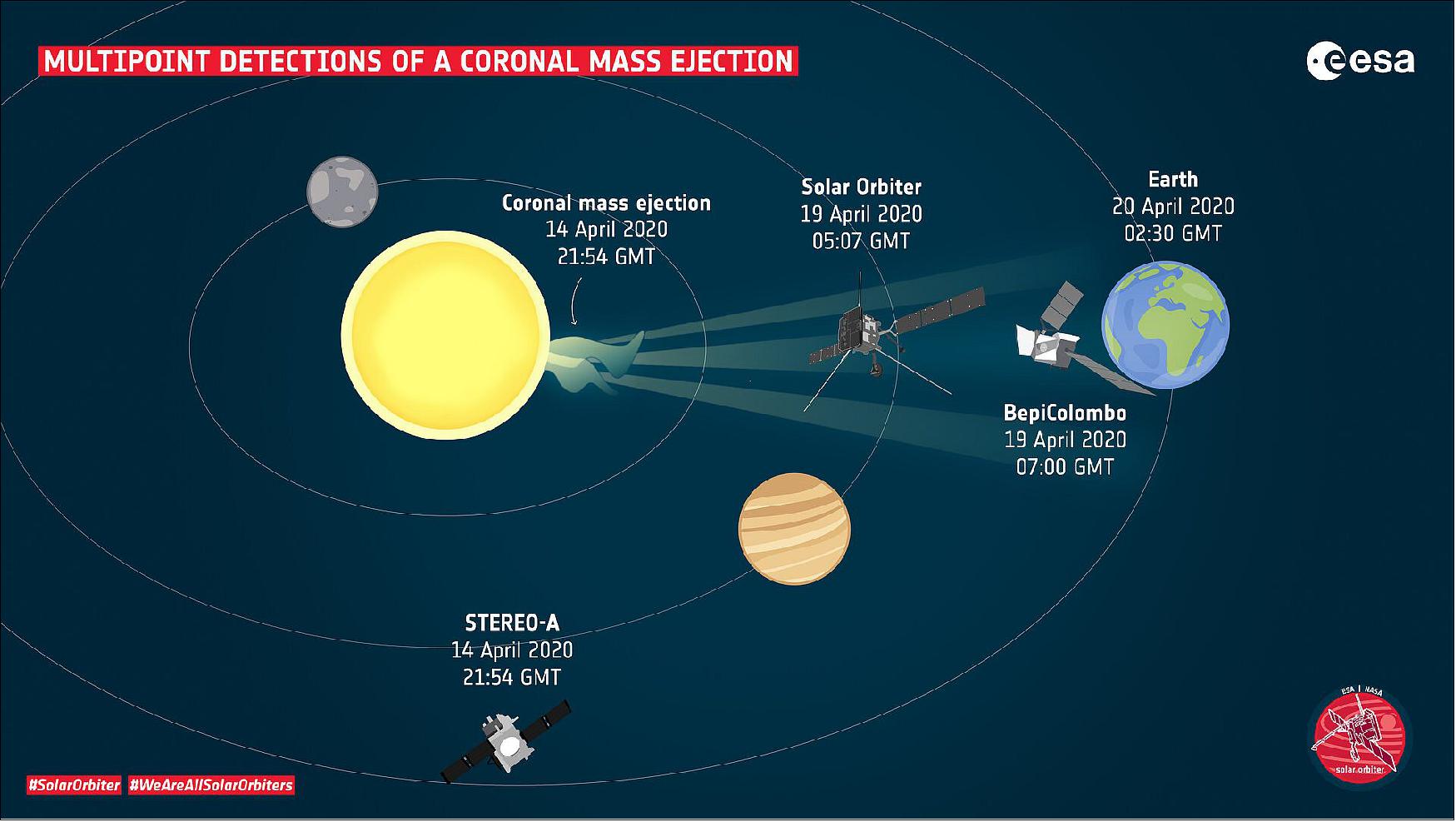
- Solar Orbiter wasn't the only spacecraft that observed this event. ESA's BepiColombo Mercury mission happened to be flying by the Earth at the time. There was also a NASA solar spacecraft called STEREO situated about ninety degrees away from the direct Sun-Earth line, and looking directly across the area of space that the CME travelled through. It watched the CME impact Solar Orbiter and then BepiColombo and Earth. Combining the measurements from all the different spacecraft allowed researchers to really study the way that the coronal mass ejection evolved as it travelled through space.
- "We can look at it remotely, we can measure it in-situ and we can see how a CME changes as it travels towards the Earth," says Tim.
- Perhaps just as intriguing as the spacecraft that saw the event, were those that didn't. The ESA-NASA SOHO spacecraft, which is situated in front of Earth and constantly watches the Sun for eruptions such as this, barely registered it. This puts the 19 April event in a rare class of space weather events, termed a stealth CME. Studying these more elusive events will help us understand space weather more completely.

- In the coming years, the opportunities for multipoint science will increase. On 27 December, Solar Orbiter will complete its first Venus flyby. This event will use the planet's gravity to swing the spacecraft closer to the Sun, putting Solar Orbiter in an even better position for joint measurements with NASA's Parker Solar Probe, which will also complete two Venus flybys in 2021.
- ESA's Solar Orbiter mission will aim to solve many open questions in solar science:
a) The Sun's surface is about 5,500ºC but the upper atmosphere – corona – is one million degrees. What causes this mysterious heating?
b) The solar wind is a stream of charged particles emitted from the Sun. The particles reach speeds of up to 800 km/s. What makes them so fast?
c) The Sun's magnetic field drives the 11-year cycle of solar activity, but what drives the Sun's magnetic field? The Sun's polar regions are key to understanding the magnetic field, but no one has ever seen them up close. Solar Orbiter will take the first images of this enigmatic region.
d)How does the Sun's magnetic field affect Earth?
- Solar Orbiter will investigate how the Sun transmits its energy across space, helping us to better understand how our local star influences our home planet in terms of space weather.
- As Parker Solar Probe of NASA makes in-situ measurements from inside the solar atmosphere, Solar Orbiter will take images of the same region. Together, the two spacecraft will give both the details and the bigger picture.
- 2021 is going to be an exciting time for Solar Orbiter," says Teresa Nieves-Chinchilla, NASA Solar Orbiter Project Scientist. "By the end of the year, all the instruments will be working together in full-fledged science mode, and we will be preparing to get even closer to the Sun."
- In 2022, Solar Orbiter will close to within 48 million km of the Sun's surface, more than 20 million km closer than it will go in 2021.
• September 30, 2020: ESA has released its first Solar Orbiter data to the scientific community and the wider public. The instruments contributing to this data release come from the suite of in-situ instruments that measure the conditions surrounding the spacecraft (see Figure 78). 58)
- As soon as a mission is in space, the teams work to commission the instruments and start collecting science data. As soon as the information starts to flow in, interest builds towards the first data release.
- In the case of many space missions, the first data release usually comes after six months or a year, to reward the teams that have built the instruments with an exclusive first look at the data. Long before launch, however, it was agreed that Solar Orbiter would be different.
- "We want Solar Orbiter to be one of the most open space missions. This means open to the whole world, not only to the teams who have built the instruments," says Yannis Zouganelis, Solar Orbiter Deputy Project Scientist for ESA.
- Based on the successful approach taken by previous solar physics missions, it was decided that the time between the data being received on Earth and it being released to the world would be at most 90 days. During this period, the instrument teams calibrate the data taken by Solar Orbiter from its ever-changing distance to the Sun. That would be a fast turn-around at the best of times; with the unprecedented challenges of the pandemic this year, it is a double achievement to hit the deadline.
- "To do this in COVID-19 times was very challenging," says Yannis, "But we are ready to deliver the data to the scientific community according to the plan, so that they can do science with it."
- The work begins long before launch, with the various instrument teams getting ready to receive and process their data. The teams themselves are composed of dozens of people, often in many different countries.
- Once the instruments are taking data, the mission enters a calibration phase in which a lot of work is put in by each instrument team to understand how their instrument is working in space, whether the data coming back is as expected, and which instrumental and spacecraft effects need to be corrected for. For example, instrument readouts depend on the temperature of the detector, but often the thermometers are by necessity located at a certain distance away. Thus, the data must be calibrated with the actual ‘in-orbit' thermal behavior of the spacecraft.
- Once the working instrument is understood, the teams process the data and send them to ESA's European Space Astronomy Centre (ESAC), near Madrid. There, the data is archived at the ESAC Science Data Centre and made accessible to the public.
- "It's a coordinated effort involving dozens of people from many different teams, many different countries, and all parties have to work together, like an orchestra, to make sure that everything is ready at the right time," says Yannis.
- This orchestra is directed by ESA's Solar Orbiter archive scientist Pedro Osuna in concert with the ESAC Science Data Centre. The effort requires dedicated hands-on commitment from all instrument teams to transform the raw data into calibrated products for scientific analysis.
- "When the data is received on the ground it is raw data, just ones and zeros," says Javier Rodríguez-Pacheco, University of Alcalá, and the Principal Investigator of EPD (Energetic Particles Detector). "This is sent to us and we transform it into physical units that can be used for scientific purposes."
- For this first data release, Javier says that most of the data was cleaned and calibrated by hand but in future, once they fully understand the responses of the different EPD sensors, they are looking to create a data pipeline that will be able to largely automate the process. Although, someone will always supervise the process.
- The data being released from RPW (Radio and Plasma Waves) comes from readings that were taken after 15 June because the period before was the commissioning phase rather than the science phase. "In the commissioning period, we do all sorts of strange things with the instrument," says Jan Soucek, Institute of Atmospheric Physics of the Czech Academy of Sciences, Prague and lead co-investigator of RPW.
- In this mode, readings are collected in a number of different ways and this makes them poorly suited for science. "If you want to look at statistics, you need to make sure that you are measuring things in a consistent way, so if we play with the instrument all the time, it's not very consistent," says Jan.
- But like the other instruments, once its behavior is known, the data can be processed relatively easily and quickly.

- In the case of MAG, the job was to learn about all the small magnetic fields that the spacecraft itself generates when its various circuits and equipment are switched on and off. Tim Horbury, Imperial College, and Principal Investigator of MAG, says that the fact the data is ready on time is testament to the hard work of the engineering team at Imperial College.
- "They have worked incredibly hard over the last few months. It's been an immense amount of work," he says. "There's a lot of it that we're releasing that nobody's really looked at in great detail yet. So I am sure there will also be a whole extra set of wonders – we just don't know what they are yet. There's an enormous amount for people to do, and I really hope that people will dive in."
- The fourth in-situ instrument, SWA (Solar Wind Analyzer), is still working on its data processing and calibration. "We have had a number of teething challenges operating safely with the high-voltages that are an integral part of all three of our sensors," says Christopher Owen, Mullard Space Science Laboratory, University College London, and SWA's principal investigator. "As a consequence we have not been able to take as much data, or to spend as much time on understanding performance as we would have liked."
- However, Chris is optimistic. "The sensors themselves are fundamentally healthy, and we can see from the data we do have that they are capable of delivering great science and fulfilling the important roles they have in delivering the unique mission science goals," he says.
- In the meantime, there is more than enough data from the other instruments for the scientific community to begin work with. In tandem with the data release, a special issue of the journal Astronomy and Astrophysics is being published that contains mission and instrument descriptions.
- "Now any scientist from any country can get the data and do science with it. In fact, there are already hundreds of scientists working together to make sense out of this unique data," says Yannis.
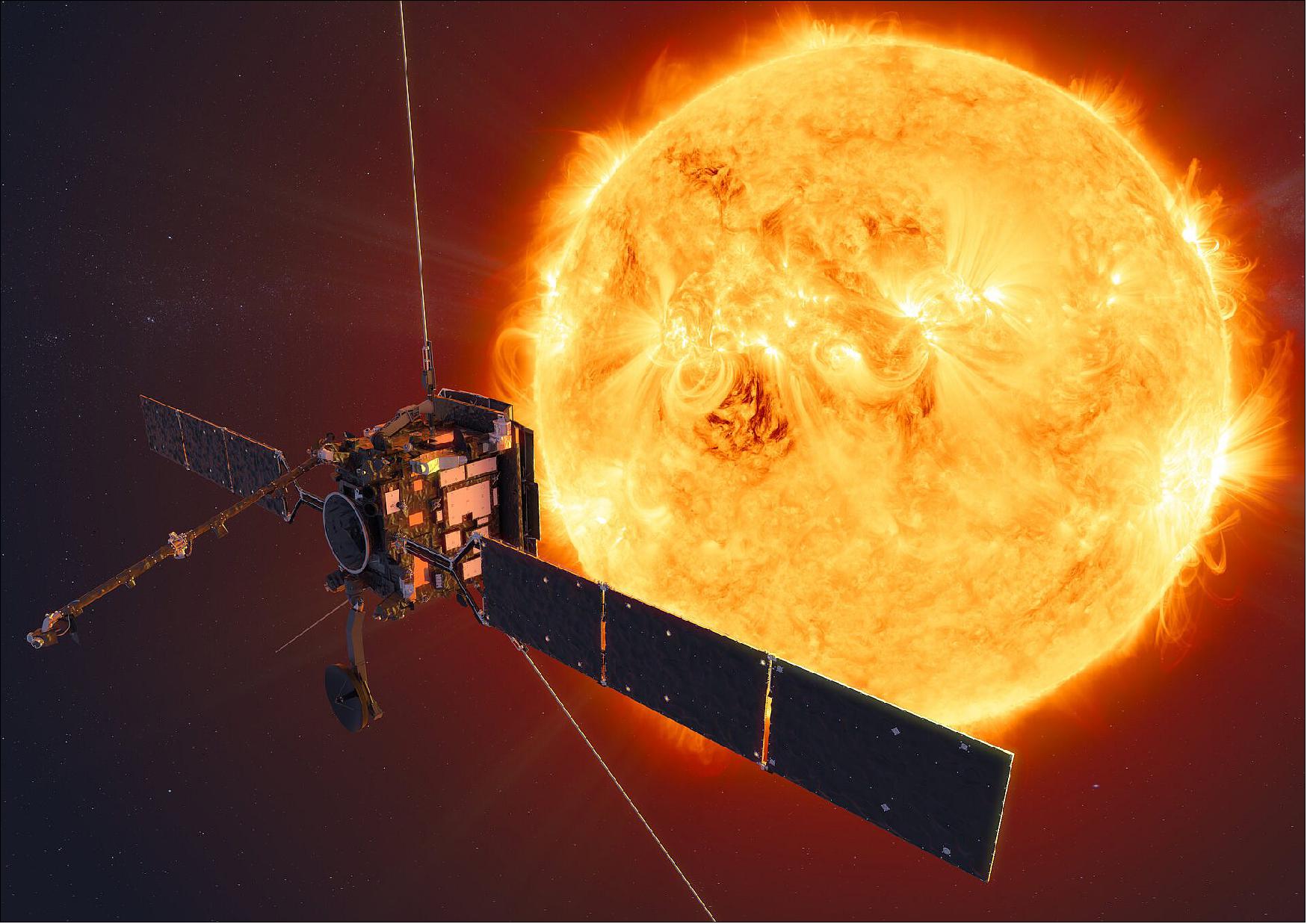
• July 16, 2020: The first images from ESA's Solar Orbiter are already exceeding expectations and revealing interesting new phenomena on the Sun. 59) 60)
- The close-up views by EUI show the upper atmosphere of the Sun, or corona, with a temperature of around 1 million degrees. With the power to see features in the solar corona of only 400 km across, these images reveal a multitude of small flaring loops, erupting bright spots and dark, moving fibrils. A ubiquitous feature of the solar surface, uncovered for the first time by these images, have been called ‘campfires'. They are omnipresent miniature eruptions that could be contributing to the high temperatures of the solar corona and the origin of the solar wind.
- The EUI images are followed by three views based on data from the PHI (Polarimetric and Helioseismic Imager) instrument. The blue and red view is a ‘tachogram' of the Sun, showing the line of sight velocity of the Sun, with the blue side turning to us and the red side turning away. The following view is a magnetogram, or a map of magnetic propertied for the whole Sun, featuring a large magnetically active region in the lower right-hand quadrant of the Sun. The yellow-orange view is a visible light image and represents what we would see with the naked eye: there are no sunspots visible because the Sun is displaying only low levels of magnetic activity at the moment.
- On larger scales, the METIS (Multi-Element Telescope for Imaging and Spectroscopy) coronagraph blocks out the dazzling light from the solar surface, bringing the fainter corona into view. METIS observes the corona simultaneously in visible light (shown in green) and ultraviolet light (shown in red) for the first time with unprecedented temporal coverage and spatial resolution. These images reveal the two bright equatorial streamers and fainter polar regions that are characteristic of the solar corona during times of minimal magnetic activity.
- On even grander scales, the Heliospheric Imager (SoloHI) telescope takes images of the solar wind – the stream of charged particles constantly released by the Sun into outer space – by capturing the light scattered by electrons in the wind. The first-light image from SoloHI is shown at the end, as a mosaic of four separate images from the instrument's four separate detectors. In this view, the Sun is located to the right of the frame, and its light is blocked by a series of baffles; the last baffle is in the field of view on the right-hand side and is illuminated by reflections from the solar array. The partial ellipse visible on the right is the zodiacal light, created by sunlight reflecting off the dust particles that are orbiting the Sun. The signal from the solar wind outflow is faint compared to the much brighter zodiacal light signal, but the SoloHI team has developed techniques to reveal it. Planet Mercury is also visible as a small bright dot near the lower edge of the upper left tile.
- Solar Orbiter is a space mission of international collaboration between ESA and NASA.
• July 16, 2020: The first images from ESA's Solar Orbiter, captured around the spacecraft's first close pass of the Sun, some 77 million kilometers from its surface, are already exceeding expectations revealing interesting new phenomena on our parent star. 61)
- Captured on 30 May 2020, when Solar Orbiter was roughly halfway between the Earth and the Sun, these are the closest views of the Sun ever taken, allowing EUI to see features in the solar corona of only 400 km across. As the mission continues, Solar Orbiter will go closer to the Sun and this will increase the instrument's resolving power by a factor of two at closest approach.
- The color on this image has been artificially added because the original wavelength detected by the instrument is invisible to the human eye.
• June 29, 2020: ESA's Solar Orbiter has successfully completed four months of painstaking technical verification, known as commissioning. Despite the challenges imposed by the COVID-19 pandemic, the spacecraft is now ready to begin performing science as it continues its cruise towards the Sun. 62)
- When Solar Orbiter blasted into space on an Atlas V rocket from Cape Canaveral, Florida, on 10 February 2020, the teams behind the €1.5 billion mission did not anticipate that within weeks, the spread of COVID-19 would evict them from their high-tech control rooms, making the challenging process of commissioning the spacecraft's instruments even harder.
- In normal circumstances, many of the project's scientists and engineers would have gathered at the European Space Operations Center (ESOC) in Darmstadt, Germany. Together, they would have worked in close cooperation with the spacecraft operators, to bring the spacecraft and its instruments to life.
- This happened more or less as usual during the most challenging early weeks of Solar Orbiter's in-orbit existence, but when the instrument teams were invited to ESOC in March, the situation in Europe was rapidly changing.
- Each of the ten instrument teams needed many representatives on site. Two or three from each team were allowed in a dedicated Solar Orbiter control room. "The other representatives worked from a dedicated support area," says Sylvain Lodiot, ESA's Solar Orbiter Spacecraft Operations Manager. It was not unusual to have 15 or more people in the main control room working too. But within a week, it became clear that European countries were heading into lockdown and so the external teams were asked to return home. The situation became even more serious when several workers at ESOC tested positive for the virus, and the site effectively closed.
The COVID Lockdown
- "We had to protect the people," says Sylvain, whose last task before going home was to switch off all the instruments on Solar Orbiter. "It felt horrible because I didn't know when those instruments were coming back online," he says.
- One of the instrument teams most affected was the Solar Wind Analyzer (SWA) team. The solar wind, which is constantly released from the Sun, is composed of a mixture of electrically charged particles called ions, and electrons. The SWA instrument comprises three different sensors to measure the fluxes and composition of these various particle populations. Each sensor operates as a kind of ‘electrical periscope' that uses high voltages, up to 30 kilovolts in one case, to divert the solar wind particles into the detector.
- To operate those high voltages safely, the team had planned not to turn on the instrument until at least a month after launch. This was intended so no traces of Earth's atmosphere would remain within the SWA sensors. If there were, these high voltages could cause arcing and damage the sensors.
- The switch on process for each of the SWA detectors is long because each high-voltage subsystem must be powered up in steps of just 20 or 50 volts at a time. After each increase, the instrument is checked to make sure nothing untoward has happened.
- When SWA's principal investigator Christopher Owen, of the Mullard Space Science Laboratory, University College London (MSSL/UCL), had left Germany, he and his team had begun to make plans to commission the instrument from their lab in the UK. But then the UK lockdown was announced, meaning a move to working from the home office for almost everybody.
- "When I left the lab, I grabbed a couple of laptops and four screens, and brought them home. I then evicted my two-year-old from his nursery and set everything up in there," says Christopher. And from this temporary control center, once ESOC had returned to work, he worked remotely with the rest of the SWA team and the skeleton staff in Darmstadt to get the instrument commissioned.
- "We had serious doubts about whether we could work like this," says Sylvain about the process in general, "but we adapted and in the end, it worked very well because the team all knew each other."
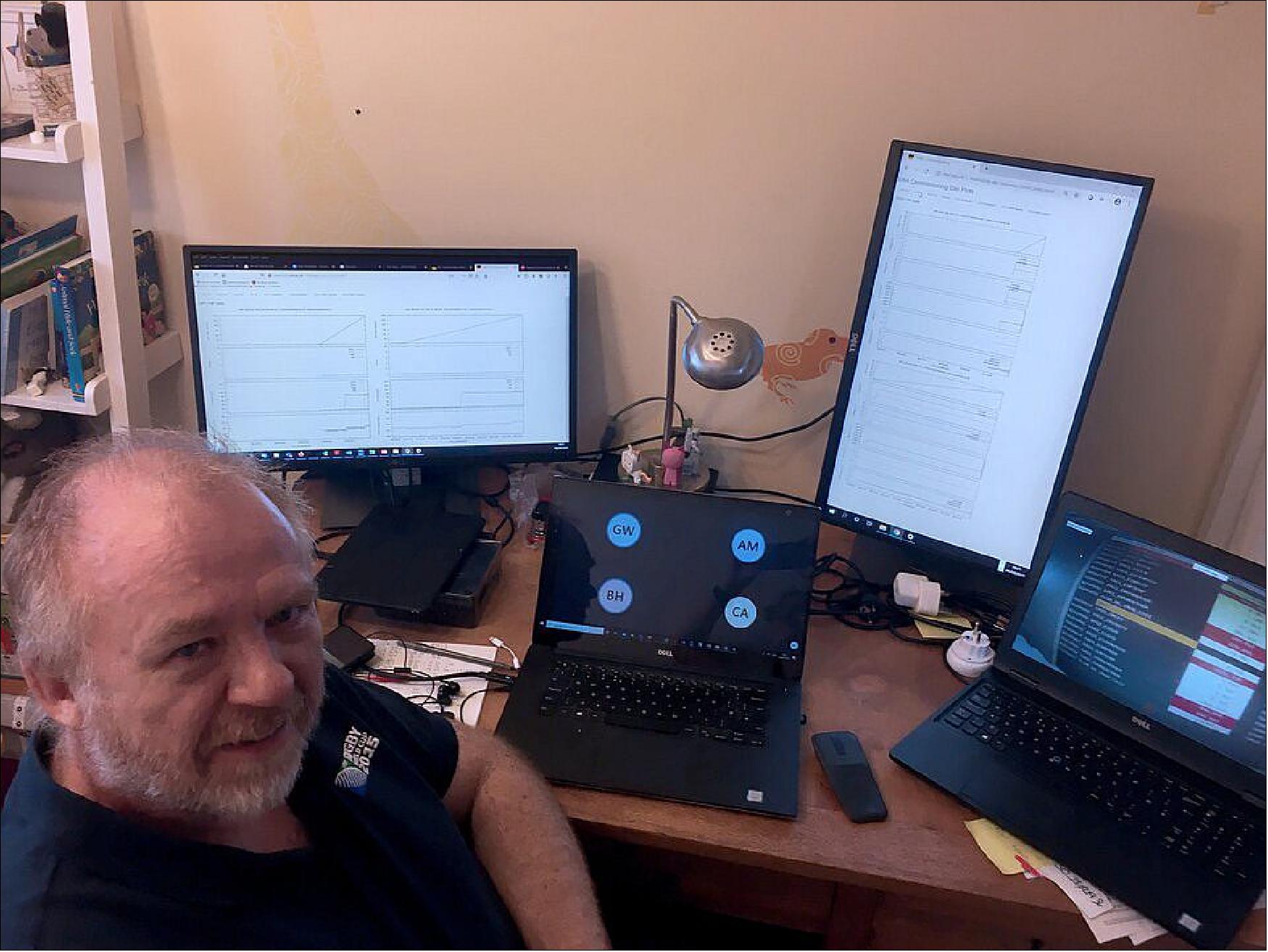
- The other instrument teams also successfully finished their commissioning. "This is undoubtedly the first mission whose instruments were completely commissioned from people's homes," says David Berghmans, from the Royal Observatory of Belgium, Brussels, Belgium, and principal investigator of the Extreme Ultraviolet Imager (EUI).
- Not only did the job get done, but they made up for lost time and managed to complete their commissioning on the original timeline. "Even in a normal world I would be very happy with where we are now," says Daniel Müller, Solar Orbiter Project Scientist at ESA, "I never expected that almost everything would work flawlessly out of the box."
- That's testimony to the expertise with which the spacecraft was made by the prime contractor Airbus DS (UK) and its instruments were made by the various instrument teams. On 25 June, the Solar Orbiter Review Board endorsed this achievement by declaring the Mission Commissioning Results Review successful.
- For César García Marirrodriga, ESA's Solar Orbiter's Project Manager, it was a big moment because with commissioning over, his job is done and he hands over the spacecraft to the mission operations manager. "I'm very happy to hand it over because I know it is going in the right direction," says César.
- And for Daniel, it is a big moment too because now the mission is ready to perform science. "In these four months since launch, the 10 instruments onboard have been carefully checked and calibrated one by one, like tuning individual musical instruments. And now it is time for them to perform together," he says.
- This month's 'remote-sensing checkout window' from 17 to 22 June presented the first opportunity to have all the instruments play together. Receiving the recordings from the spacecraft, which is currently more than 160 million km away, will be completed in the next few days.
- "We're very excited about this first ‘concert'. For the first time, we will be able to put together the images from all our telescopes and see how they take complementary data of the various parts of the Sun including the surface; the outer atmosphere, or corona; and the wider heliosphere around it. This is what the mission was built for," says Daniel. These first light images will be released to the public in mid-July.
- Other instruments are already collecting data too. In the case of the Magnetometer (MAG), this was first switched on just a day after launch. "We got just under 100 days' worth of data through the commissioning period, and it's wonderful data," says Helen O'Brien, from Imperial College and MAG's chief engineer.
- MAG was switched on early so that it could take readings as it was carried away from the spacecraft as its boom arm was deployed. "The instrument behaved beautifully. It was wonderful to see the field decay as we moved away from the spacecraft," says Helen.
- That data will allow the team to understand the magnetic field being generated by the spacecraft itself, so that they can now remove it from their science data to leave just the magnetic field being carried into space away from the Sun. And there is plenty of data already. The team already has more than two billion scientific measurements to analyze. "The data is outstanding, really, really good, so we're very happy," says Tim Horbury, Imperial College, UK, and principal investigator for the instrument.
- The mission now continues on course to the Sun. During this cruise phase, the spacecraft's in-situ instruments will gather scientific data about the environment around the spacecraft, while the remote-sensing instruments will be fine-tuned by the teams in preparation for science operations in closer vicinity of the Sun. The cruise phase lasts until November 2021, after which Solar Orbiter will begin the science phase of its mission.
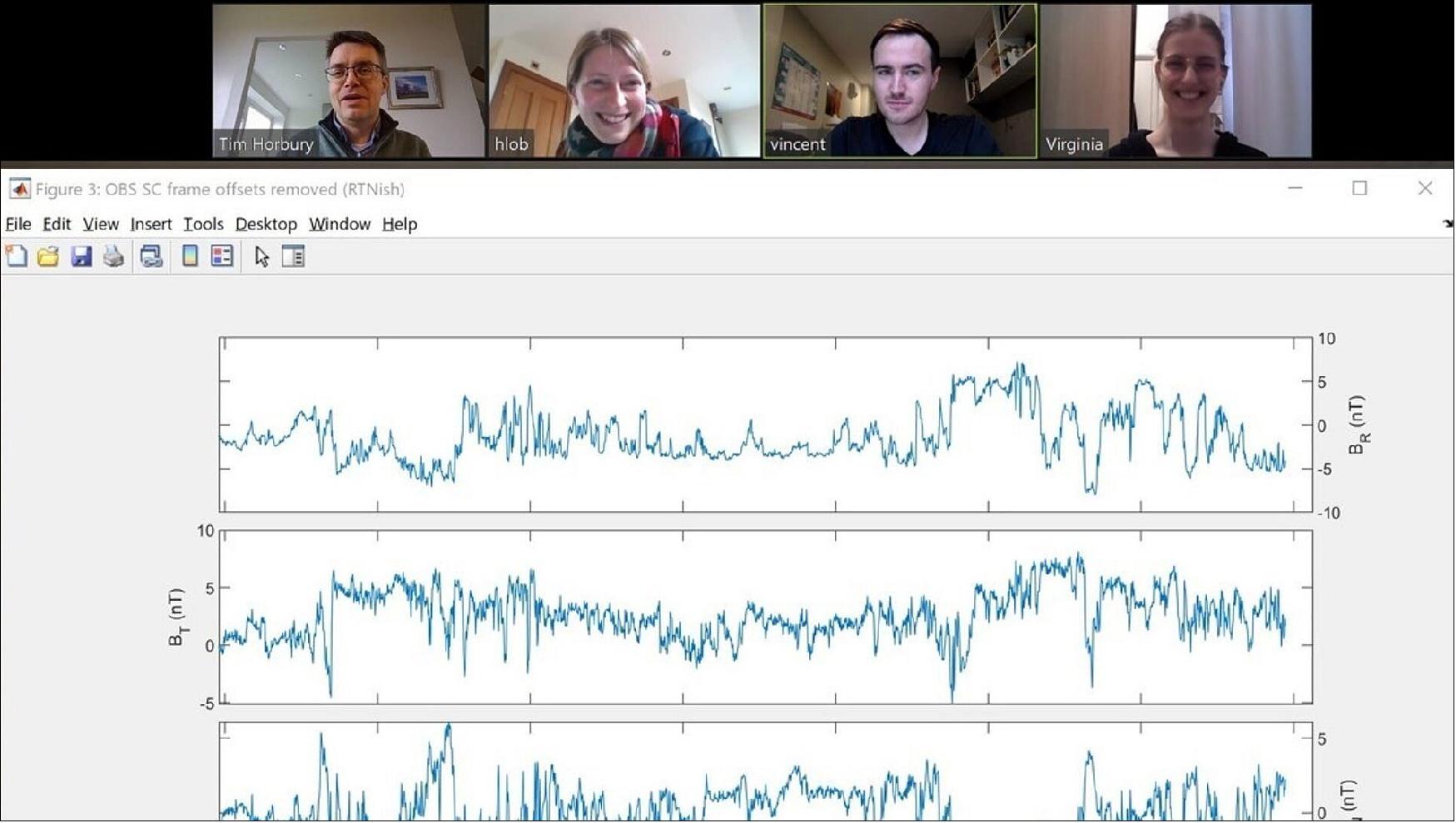
• June 15, 2020: ESA's Sun-exploring mission Solar Orbiter has made its first close approach to the star on June 15, getting as close as 77 million km to its surface, about half the distance between the Sun and Earth. 63)
- In the week following this first perihelion, the point in the orbit closest to the Sun, the mission scientists will test the spacecraft's ten science instruments, including the six telescopes on-board, which will acquire close-up images of the Sun in unison for the first time. According to ESA's Solar Orbiter Project Scientist Daniel Müller, the images, to be released in mid-July, will be the closest images of the Sun ever captured.
- "We have never taken pictures of the Sun from a closer distance than this," Daniel says. "There have been higher resolution close-ups, e.g. taken by the four-meter Daniel K. Inouye Solar Telescope in Hawaii earlier this year. But from Earth, with the atmosphere between the telescope and the Sun, you can only see a small part of the solar spectrum that you can see from space."
- NASA's Parker Solar Probe, launched in 2018, makes closer approaches. The spacecraft, however, doesn't carry telescopes capable of looking directly at the Sun.
- "Our ultraviolet imaging telescopes have the same spatial resolution as those of NASA's Solar Dynamic Observatory (SDO), which takes high-resolution images of the Sun from an orbit close to Earth. Because we are currently at half the distance to the Sun, our images have twice SDO's resolution during this perihelion," says Daniel.
- The primary objective of these early observations is to prove that Solar Orbiter's telescopes are ready for future scientific observations.
- "For the first time, we will be able to put together the images from all our telescopes and see how they take complementary data of the various parts of the Sun including the surface, the outer atmosphere, or corona, and the wider heliosphere around it," says Daniel.
- The scientists will also analyze data from the four in-situ instruments that measure properties of the environment around the spacecraft, such as the magnetic field and the particles making up the solar wind.
- "This is the first time that our in-situ instruments operate at such a close distance to the Sun, providing us with a unique insight into the structure and composition of the solar wind," says Yannis Zouganelis, ESA's Solar Orbiter Deputy Project Scientist. "For the in-situ instruments, this is not just a test, we are expecting new and exciting results."
- Solar Orbiter, launched on 10 February this year, is completing its commissioning phase on 15 June and will commence its cruise phase, which will last until November 2021. During the main science phase that follows, the spacecraft will get as close as 42 million km to the Sun's surface, which is closer than the planet Mercury.
- The spacecraft will reach its next perihelion in early 2021. During the first close approach of the main science phase, in early 2022, it will get as close as 48 million km.
- Since the spacecraft is currently 134 million km from Earth, it will take about a week for all perihelion images to be downloaded via ESA's 35-m deep-space antenna in Malargüe, Argentina. The science teams will then process the images before releasing them to the public in mid-July. The data from the in-situ instruments will become public later this year after a careful calibration of all individual sensors.
- "We have a nine-hour download window every day but we are already very far from Earth so the data rate is much lower than it was in the early weeks of the mission when we were still very close to Earth," says Daniel. "In the later phases of the mission, it will occasionally take up to several months to download all the data because Solar Orbiter really is a deep space mission. Unlike near-Earth missions, we can store a lot of data on-board and downlink it when we are closer to home again and the data connection is much better."
• May 29, 2020: ESA's Solar Orbiter will cross through the tails of Comet ATLAS during the next few days. Although the recently launched spacecraft was not due to be taking science data at this time, mission experts have worked to ensure that the four most relevant instruments will be switched on during the unique encounter. 64)
- Solar Orbiter was launched on 10 February 2020. Since then, and with the exception of a brief shutdown due to the coronavirus pandemic, scientists and engineers have been conducting a series of tests and set-up routines known as commissioning.
- The completion date for this phase was set at 15 June, so that the spacecraft could be fully functional for its first close pass of the Sun, or perihelion, in mid-June. However, the discovery of the chance encounter with the comet made things more urgent.
- Serendipitously flying through a comet's tail is a rare event for a space mission, something scientists know to have happened only six times before for missions that were not specifically chasing comets. All such encounters have been discovered in the spacecraft data after the event. Solar Orbiter's upcoming crossing is the first to be predicted in advance.
- It was noticed by Geraint Jones of the UCL Mullard Space Science Laboratory, UK, who has a 20-year history of investigating such encounters. He discovered the first accidental tail crossing in 2000, while investigating a strange disturbance in data recorded by the ESA/NASA Ulysses Sun-studying spacecraft in 1996. This study revealed that the spacecraft had passed through the tail of Comet Hyakutake, also known as ‘The Great Comet of 1996'. Soon after the announcement, Ulysses crossed the tail of another comet, and then a third one in 2007.
- Earlier this month, realizing that Solar Orbiter was going to be 44 million kilometers downstream of Comet C/2019 Y4 (ATLAS) in just a matter of weeks, Geraint immediately alerted the ESA team.
Bonus Science
- Solar Orbiter is equipped with a suite of 10 in-situ and remote-sensing instruments to investigate the Sun and the flow of charged particles it releases into space – the solar wind. Fortuitously, the four in-situ instruments are also perfect for detecting the comet's tails because they measure the conditions around the spacecraft, and so they could return data about the dust grains and the electrically charged particles given off by the comet. These emissions create the comet's two tails: the dust tail that is left behind in the comet's orbit and the ion tail that points straight away from the Sun.
- Solar Orbiter will cross the ion tail of Comet ATLAS on 31 May–1 June, and the dust tail on 6 June. If the ion tail is dense enough, Solar Orbiter's magnetometer (MAG) might detect the variation of the interplanetary magnetic field because of its interaction with ions in the comet's tail, while the Solar Wind Analyzer (SWA) could directly capture some of the tail particles.
- When Solar Orbiter crosses the dust tail, depending on its density – which is extremely difficult to predict – it is possible that one or more tiny dust grains may hit the spacecraft at speeds of tens of kilometers per second. While there is no significant risk to the spacecraft from this, the dust grains themselves will be vaporised on impact, forming tiny clouds of electrically charged gas, or plasma, which could be detected by the Radio and Plasma Waves (RPW) instrument.
- "An unexpected encounter like this provides a mission with unique opportunities and challenges, but that's good! Chances like this are all part of the adventure of science," says Günther Hasinger, ESA Director of Science.
- One of those challenges was that the instruments seemed unlikely to all be ready in time because of the commissioning. Now, thanks to a special effort by the instrument teams and ESA's mission operations team, all four in-situ instruments will be on and collecting data, even though at certain times the instruments will need to be switched back into commissioning mode to ensure that the 15 June deadline is met.
- "With these caveats, we are ready for whatever Comet ATLAS has to tell us," says Daniel Müller, ESA Project Scientist for Solar Orbiter.
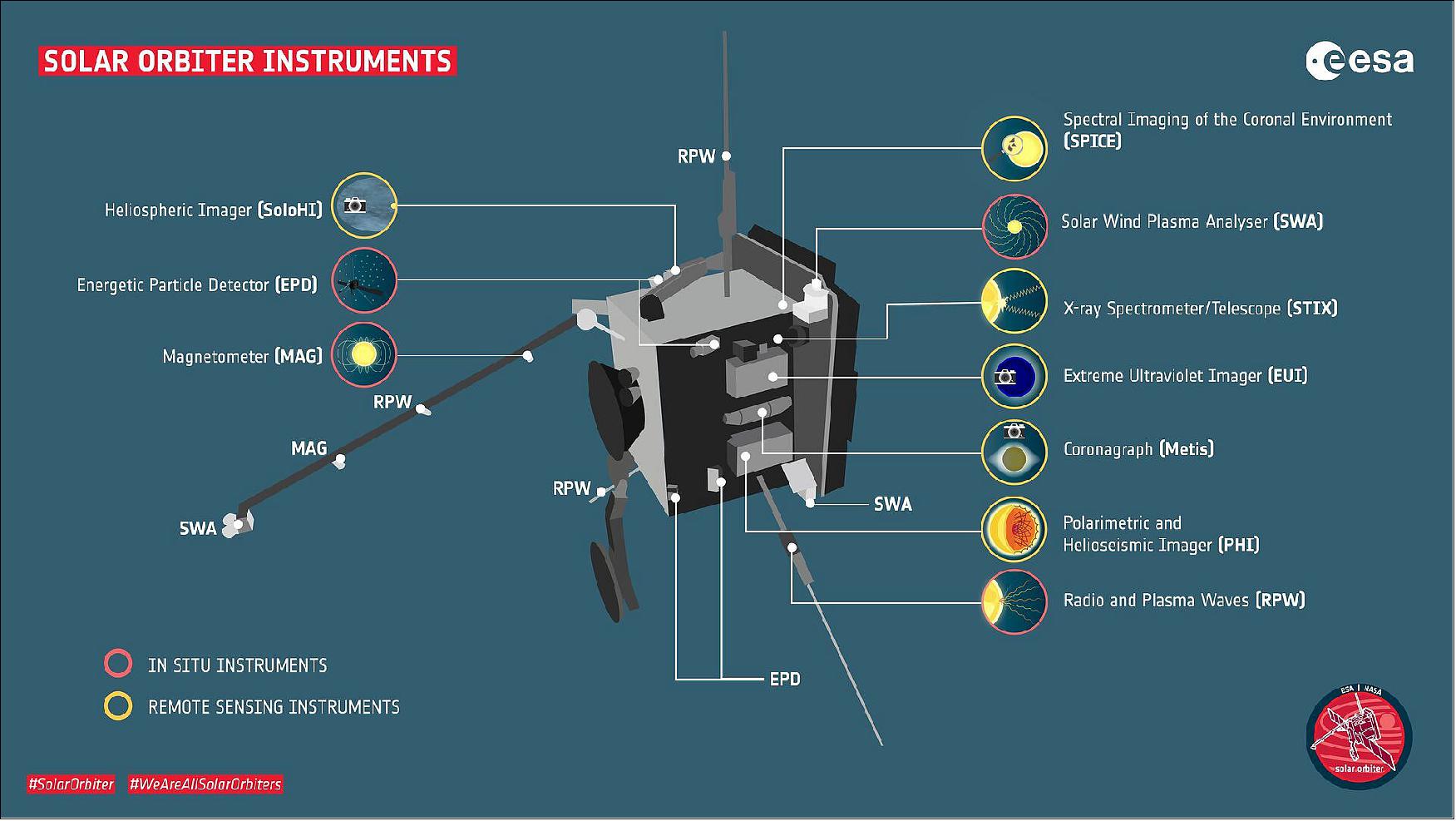
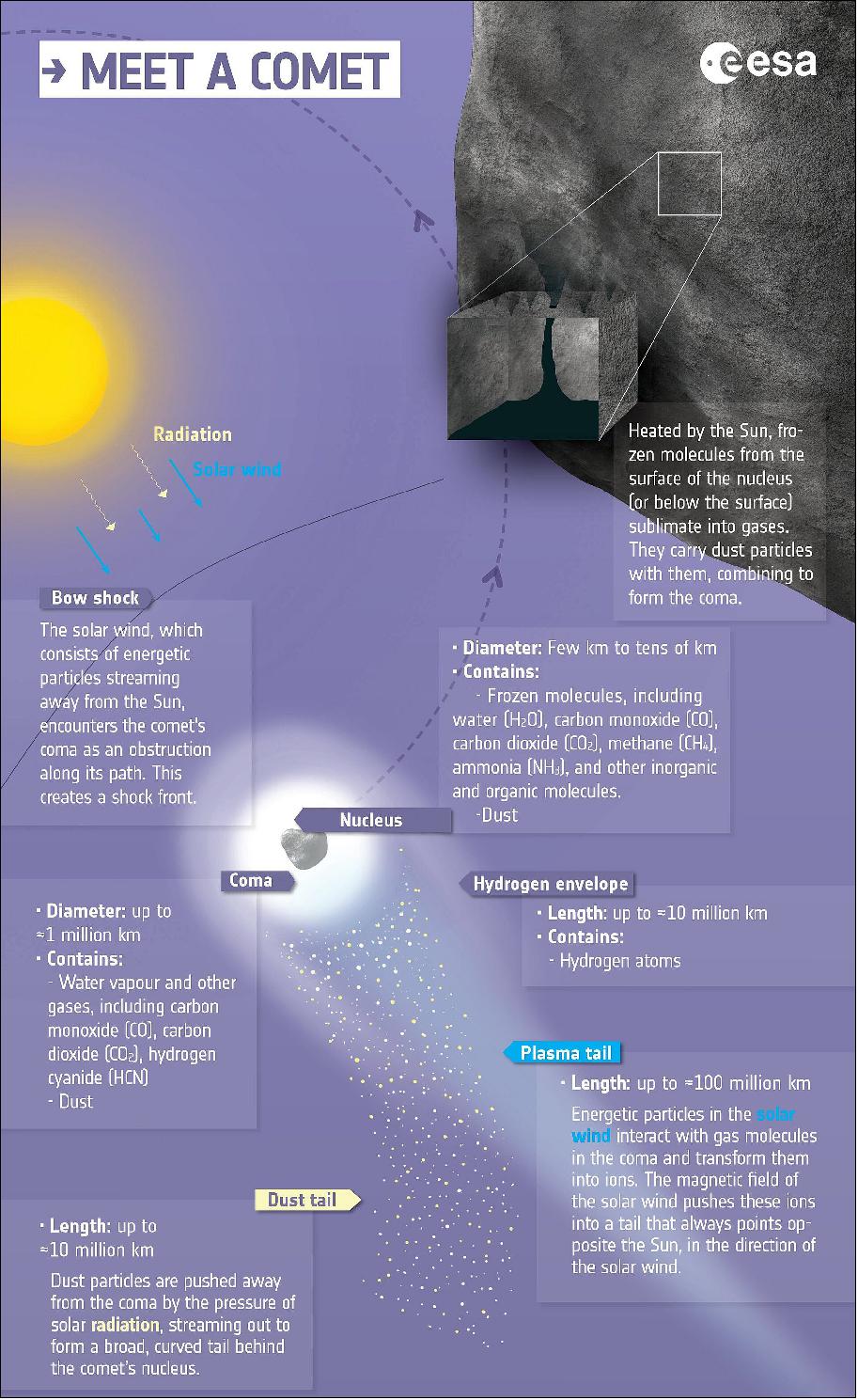
Expect the Unexpected
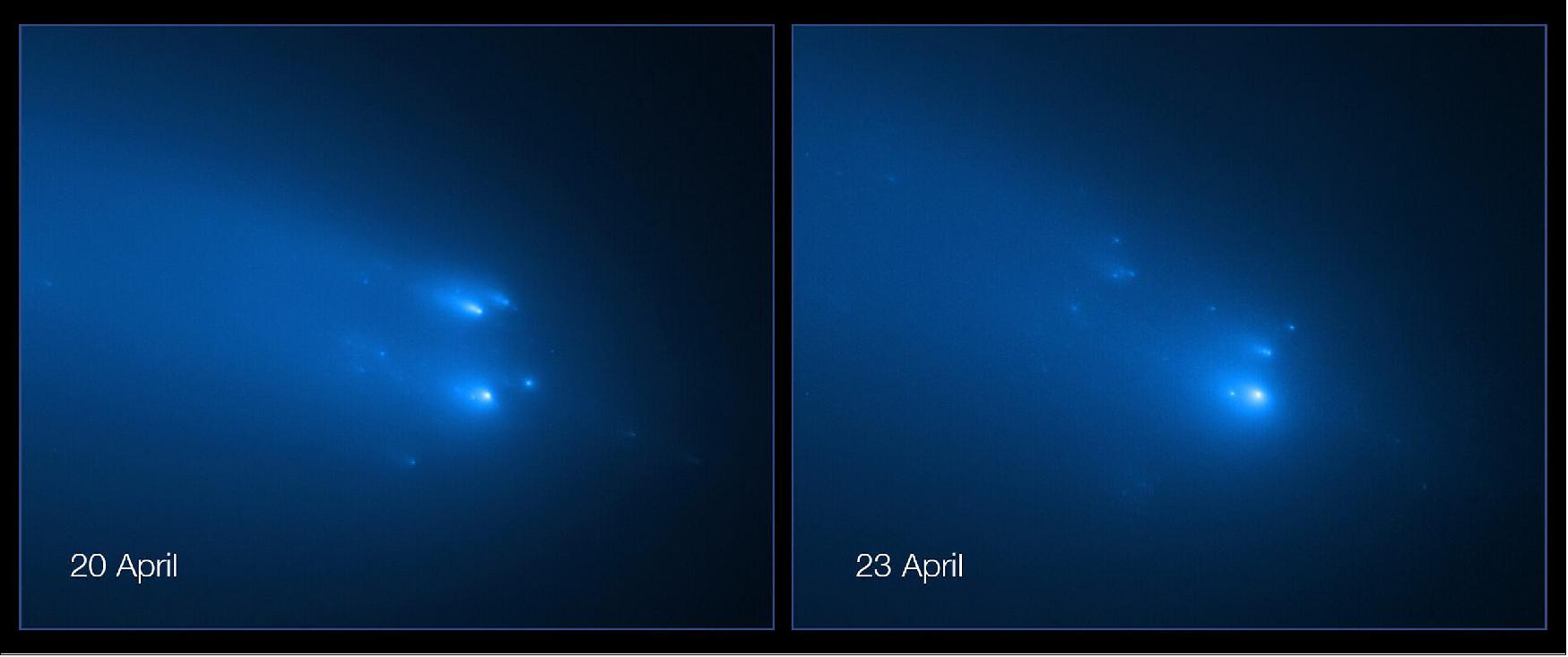
- Another challenge entails the comet's behavior. Comet ATLAS was discovered on 28 December 2019. During the next few months, it brightened so much that astronomers wondered whether it would become visible to the naked eye in May.
- Unfortunately, in early April the comet fragmented. As a result, its brightness dropped significantly too, robbing sky watchers of the view. A further fragmentation in mid-May has diminished the comet even more, making it less likely to be detectable by Solar Orbiter.
- Although the chances of detection have reduced, the effort is still worth making according to Geraint.
- "With each encounter with a comet, we learn more about these intriguing objects. If Solar Orbiter detects Comet ATLAS's presence, then we'll learn more about how comets interact with the solar wind, and we can check, for example, whether our expectations of dust tail behavior agree with our models," he explains. "All missions that encounter comets provide pieces of the jigsaw puzzle."
- Geraint is the principal investigator of ESA's future Comet Interceptor mission, which consists of three spacecraft and is scheduled for launch in 2028. It will make a much closer flyby of an as yet unknown comet that will be selected from the newly discovered comets nearer the time of launch (or even after that).
Grazing the Sun
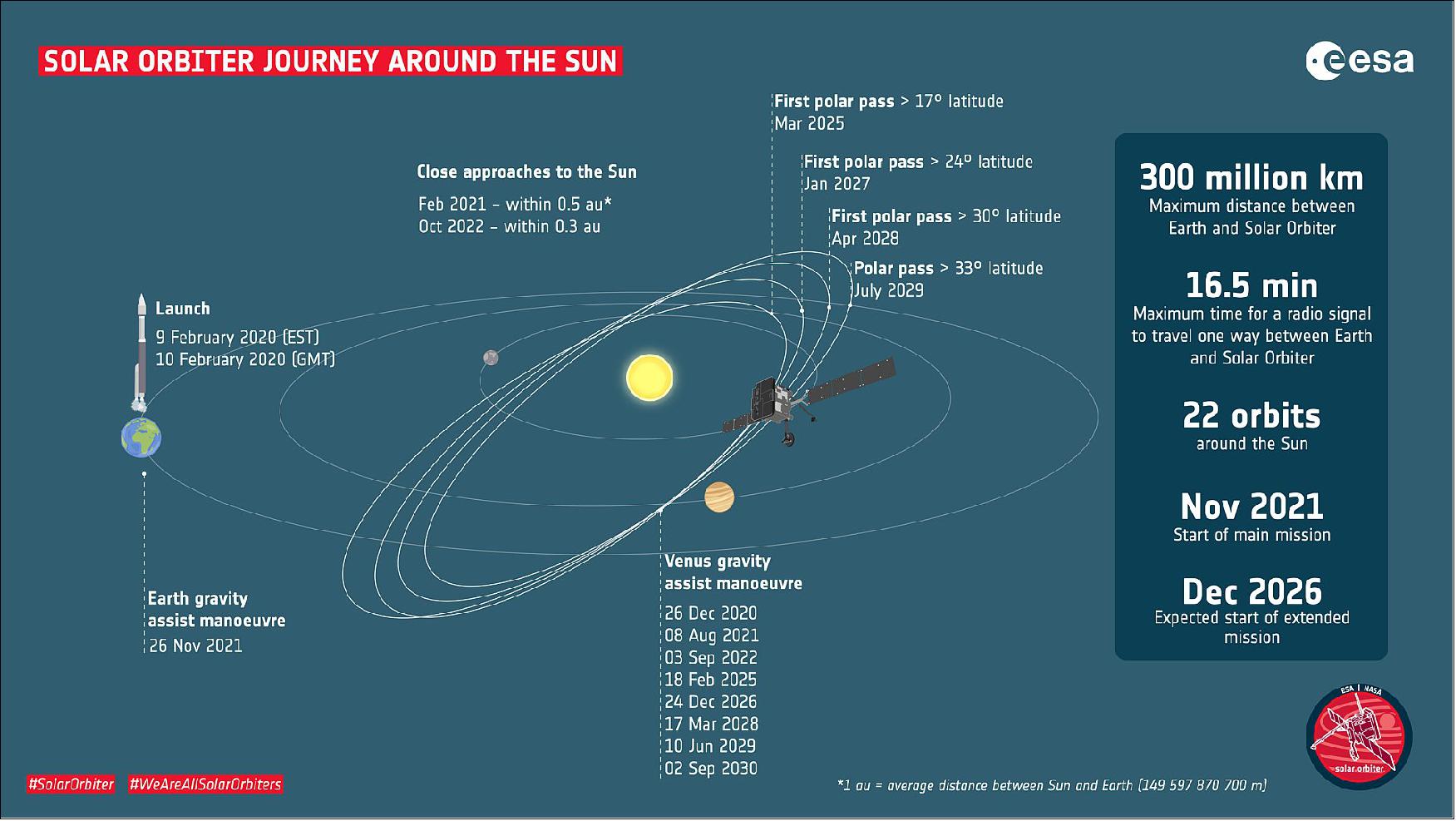
- Solar Orbiter is currently circling our parent star between the orbits of Venus and Mercury, with its first perihelion to take place on 15 June, around 77 million kilometers from the Sun. In coming years, it will get much closer, within the orbit of Mercury, around 42 million kilometers from the solar surface. Meanwhile, Comet ATLAS is already there, approaching its own perihelion, which is expected on 31 May, around 37 million kilometers from the Sun.
- "This tail crossing is also exciting because it will happen for the first time at such close distances from the Sun, with the comet nucleus being inside the orbit of Mercury," says Yannis Zouganelis, ESA Deputy Project Scientist for Solar Orbiter.
- Understanding the dust environment in the innermost region of the Solar System is one of Solar Orbiter's scientific objectives.
- "Near-Sun comets like Comet ATLAS are sources of dust in the inner heliosphere and so this study will not only help us understand the comet, but also the dust environment of our star," adds Yannis.
- Looking at an icy object rather than the scorching Sun is certainly an exciting – and unexpected – way for Solar Orbiter to start its scientific mission, but that's the nature of science.
- Scientific discovery is built on good planning and serendipity. In the three months since launch, the Solar Orbiter team has already proved that it's ready for both," says Daniel.
• February 17, 2020: First measurements by a Solar Orbiter science instrument reached the ground on Thursday 13 February providing a confirmation to the international science teams that the magnetometer on board is in good health following a successful deployment of the spacecraft's instrument boom. 65)
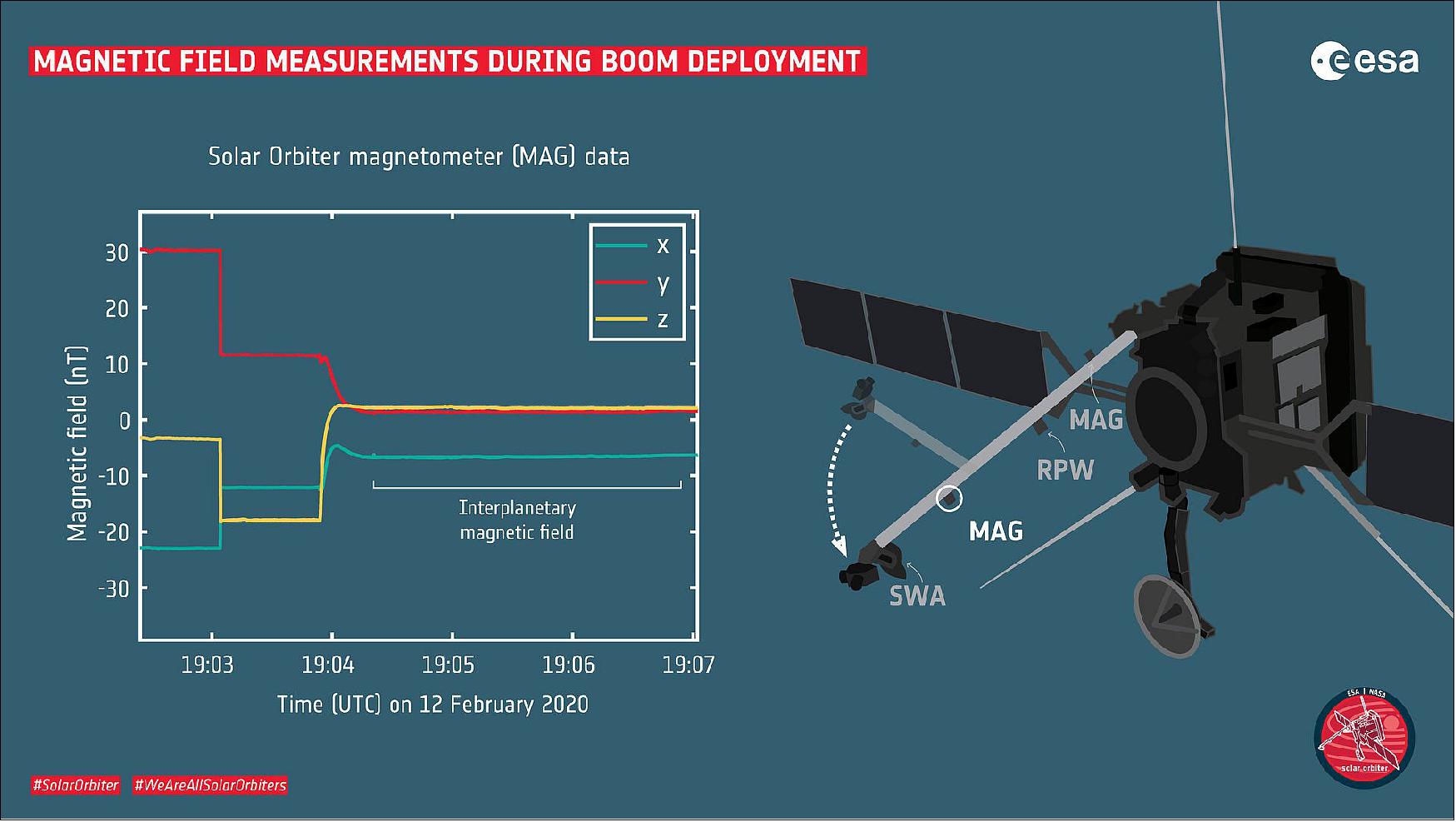
- Ground controllers at ESOC in Darmstadt, Germany, switched on the magnetometer's two sensors (one near the end of the boom and the other close to the spacecraft) about 21 hours after liftoff. The instrument recorded data before, during and after the boom's deployment, allowing the scientists to understand the influence of the spacecraft on measurements in the space environment.
- "We measure magnetic fields thousands of times smaller than those we are familiar with on Earth," says Tim Horbury of Imperial College London, Principal Investigator for the Magnetometer instrument (MAG). "Even currents in electrical wires make magnetic fields far larger than what we need to measure. That's why our sensors are on a boom, to keep them away from all the electrical activity inside the spacecraft."
- "The data we received shows how the magnetic field decreases from the vicinity of the spacecraft to where the instruments are actually deployed," adds Tim. "This is an independent confirmation that the boom actually deployed and that the instruments will, indeed, provide accurate scientific measurements in the future."
- As the titanium/carbon-fibre boom stretched out over an overall 30-minute period on Wednesday, almost three days after liftoff, the scientists could observe the level of the magnetic field decrease by about one order of magnitude. While at the beginning they saw mostly the magnetic field of the spacecraft, at the end of the procedure, they got the first glimpse of the significantly weaker magnetic field in the surrounding environment.
- "Measuring before, during, and after the boom deployment helps us to identify and characterize signals that are not linked to the solar wind, such as perturbations coming from the spacecraft platform and other instruments," says Matthieu Kretzschmar, of Laboratoire de Physique et Chimie de l'Environnement et de l'Espace in Orleans, France, Lead Co-investigator behind another sensor located on the boom, the high frequency magnetometer of the Radio and Plasma Waves instrument (RPW) instrument.
- "The spacecraft underwent extensive testing on ground to measure its magnetic properties in a special simulation facility, but we couldn't fully test this aspect until now, in space, because the test equipment usually prevents us from reaching the needed very low level of magnetic field fluctuations," he adds.
• February 14, 2020: At 16:00 CET on Thursday, 13 February, the critical first 83 hours of Solar Orbiter's unique mission to study our star came to an end. 66)
- "This early phase is like the birth of a child," says Operations Director Andrea Accomazzo. "Engineers want to be sure that it can survive on its own in its new environment."
- "In the case of a spacecraft, they need it to be powered by its solar arrays, able to communicate with Earth, and able to control its orientation in space."
- After a thunderous launch at 05:03 CET on Monday, Solar Orbiter headed south east from Cape Canaveral Air Force Station in Florida, flying over the South Atlantic before being released far above the shores of Western Australia. In Darmstadt, ESA's mission control team soon took control of the spacecraft and began several days of round-the-clock flight control activities.
- "We planned for years and trained for months for this and I'm proud of all the teams that worked through this critical period," says Andrea. "The first stage of Solar Orbiter's mission was a success, but it certainly threw us a few challenging moments!"
Sunny Side Up
- The solar arrays were deployed at 06:24 CET and unfolded from the spacecraft's body like wings. They soon began to supply power to the craft, relieving the burden on the batteries.
- With communication established and the necessary tests carried out, the first command was sent to the craft and the mission officially began.
- Until then, Solar Orbiter had still been in its strobing phase – rotating to maximize the chance of pointing one of its two communication antennas at ground stations on Earth. With this command, the spacecraft fired its thrusters and stabilized its orientation.

Rise and shine
- The mission control team then began to wake up vital parts of the craft. They switched on the ‘reaction wheels' – used to more precisely control the orientation of the spacecraft than can be achieved with thrusters – and the ‘Mass Memory' to store the science data collected by Solar Orbiter's science instruments.
- "Following successful tests, Solar Orbiter was brought into its Nominal Control Mode," says Andrea.
- "Then came the window for the deployment of the ‘Radio and Plasma Waves' (RPW) antennas, the instrument boom, and the high-gain antenna. The antennas and the boom are used to move sensors away from the spacecraft body to prevent disturbances to their measurements, while the high-gain antenna is used to communicate with the spacecraft across astronomical distances."
Cool Under Pressure
- Before each antenna or boom could be deployed, Solar Orbiter was tilted to point it towards the Sun and warm it up. After the deployment of the first RPW antenna, the instrument boom was due next.
- But as the team tilted the craft to warm up the instrument boom, they noticed something unexpected.
- "We saw that the pins that hold the doors of the remote observation instruments safely in place during launch were cooling down more rapidly than expected as we tilted them away from the Sun," says Andrea.
- "If their temperature had fallen to below -40°C, they could have undergone ‘cold welding', sticking them in place and preventing the doors of the remote sensing instruments from opening."
- "The pins had already gotten too cold to move, and were in danger of sticking. To prevent this, we sent the command for Solar Orbiter to enter its ‘safe mode', resetting its orientation and pointing the pins back towards the Sun."
- "Every launch comes with a unique set of challenges. But our teams train for events like this and were able to quickly respond to this situation and ensure a safe start to Solar Orbiter's mission."
Out with the Boom
- Once warm enough, the pins were safely moved (it had now proven necessary to move them at an earlier stage than originally planned) and the team continued with the deployment of the boom.
- Following the deployment of the second and third RPW antennas and the high-gain antenna, operations began to wind down as the radio communication link between Solar Orbiter and ESA's ground stations was switched to the more powerful high-gain antenna.
- "We were thrilled to see that the instrument boom and all three electric antennas were correctly deployed," says Yannis Zouganelis, ESA's deputy project scientist for the Solar Orbiter mission.
- "These appendages will enable us to probe the solar wind and, together with the remote-sensing instruments, reveal our Sun and its behavior in unprecedented detail. We can't wait to start taking our measurements."
- Solar Orbiter will now spend approximately three months in its commissioning phase, during which the mission control teams will conduct a test maneuver and check that the spacecraft and its 10 scientific instruments are working as intended to achieve the mission's ambitious goals.
- In roughly two years, Solar Orbiter will reach its primary science orbit where it will study our star's polar regions like no spacecraft has before.
• February 11, 2020: Solar Orbiter is ESA's latest mission to study the Sun up close. Launched in the early hours of 10 February from Cape Canaveral, Florida, the spacecraft is due to arrive at its fiery destination in approximately two years. 67)
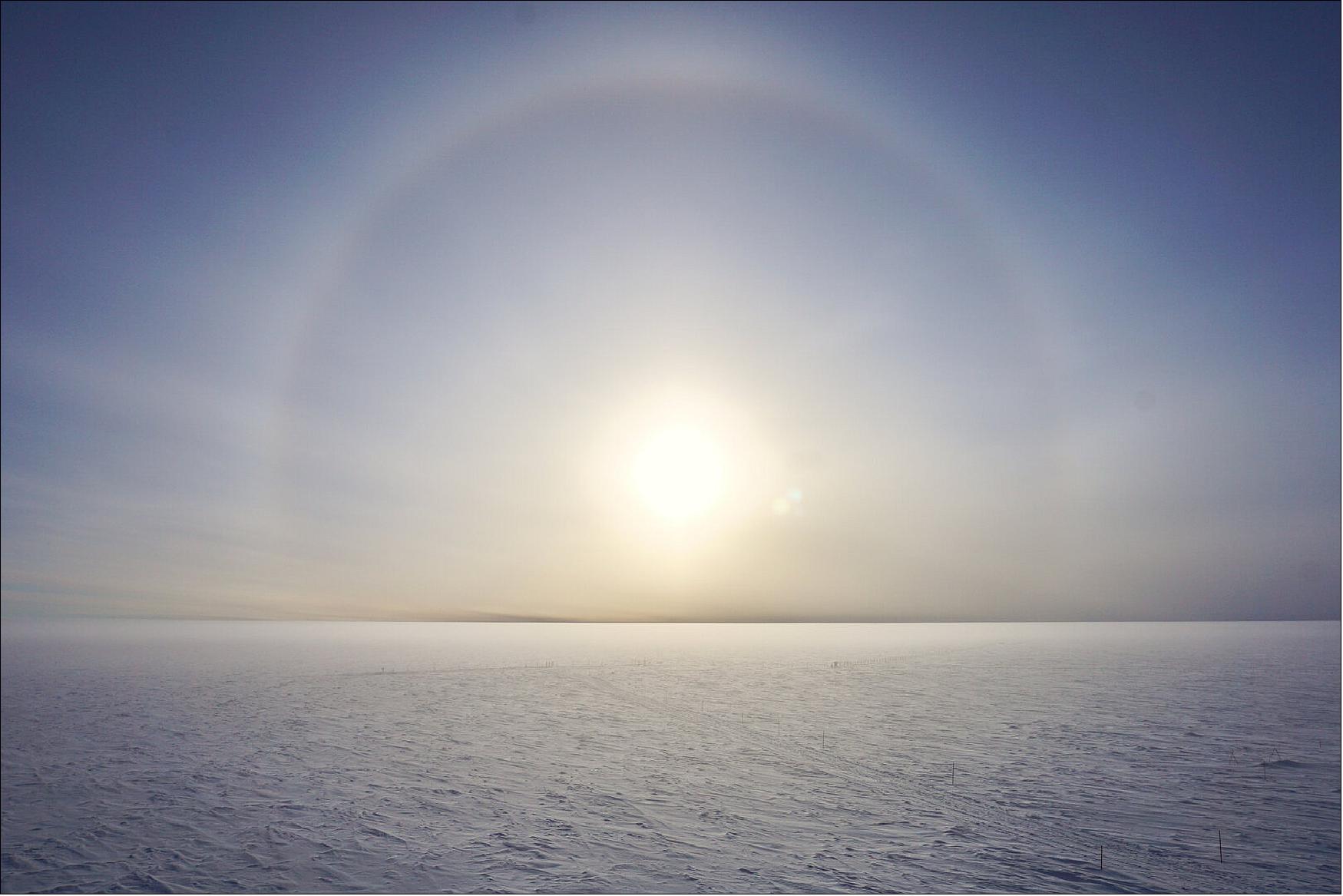
- Solar Orbiter will face the Sun from within the orbit of Mercury, approximately 42 million kilometers from the solar surface. This is an ideal distance: from here Solar Orbiter can take remote images and measurements that will provide the first views of the Sun's uncharted polar regions.
- At the southern poles on Earth, in Antarctica, the Sun has an exceptional presence on people living at the remote Concordia research station. During the Antarctic summer, the sun shines 24 hours a day. It would be perfect for sunbathing, except for the fact that the average summer temperature is only –30ºC.
- Consequently, in the winter the Sun does not appear above the horizon for over three months and the crew stationed in Concordia live with outside temperatures of –80ºC in complete darkness.
- While Solar Orbiter is en route to observing the Sun up close, the crew in Concordia are preparing for life without and enjoying the last rays of sunlight while they can. This picture shows a halo that can occur when sunlight is refracted off ice crystals in the atmosphere.
- The mission will investigate how intense radiation and energetic particles being blasted out from the Sun and carried by the solar wind through the Solar System impact our home planet, to better understand and predict periods of stormy ‘space weather'.
- While this results in beautiful aurora seen in the Arctic and Antarctic circles, stormy space weather can be disastrous. Solar storms have the potential to knock out power grids, disrupt air traffic and telecommunications, and endanger space-walking astronauts, for example.
- A better understanding of how our parent star works is critical to our preparedness for these scenarios on Earth.
Sensor Complement
The instruments have been selected jointly by ESA and NASA as part of the collaboration to provide the in situ and remote observations. The sensor complement consists of six remote sensing instruments operating at wavelength ranges from visible to X-ray, as well as four in-situ instruments covering all attributes of the interplanetary medium. It will acquire simultaneous spectra and images of the photosphere and corona; images of the photospheric magnetic field and gas velocity as well as measurements of the magnetic field and in-situ plasma properties at the location of the spacecraft. 68)
The challenging nature of the Solar Orbiter mission, along with tough constraints in the area of volume, mass and data rates, have led to a range of innovative design solutions for the payload, involving new technologies never previously used in space. In the thermal and optical domains, heat rejecting windows, limiting the bulk of the solar flux while allowing the wavelength(s) of interest to pass through, are being developed for two instruments. Several multi-layer coatings are also being designed for internal lenses and mirrors. For the three deployable antennas, the harsh thermal environment has had an important influence on the design of the deployment mechanism. For the purpose of polarization measurements, newly space qualified Liquid Crystal Variable Retarder technology is being applied and several instruments are also making advances in detector design, including newly designed CdTe X-ray detectors and back-illuminated Extreme UV CMOS detectors.
The remote sensing instruments opto-mechanical assemblies are mounted at the periphery of the main structure, by means of isostatic mounts thermally insulating the instruments from the rest of the spacecraft. This technique permits a direct FOV for the instruments and their baffle to cold space behind the heatshield, while keeping the main structure unaffected by the thermal control of the instruments; hence, insuring the very stable thermoelastic behavior needed for the line of sight (LOS) co-alignment.
Instrument | Pointing direction & FOV | LOS pointing stability (RPE) | Instrument accommodation | Institution and PI (Principal Investigator) | No of units |
Remote sensing instruments | |||||
PHI | Sun pointing | 0.02 arcsec in 10 s | Located behind shield, 2 apertures (12.5 and 1.5 cm diameter) with door and heat rejection filters | MPS (Max Planck Institute for Solar System Research), Lindau, Germany, PI: Sami Solanki | 2(+2 windows) |
SPICE | Sun pointing | 1.0 arcsec in 10 s | Located behind shield, 1 aperture (7 cm diameter) with door | RAL Space, UK (ESA funded) | 2 |
EUI | Sun pointing | 1.0 arcsec in 10 s | Located behind shield, 4 apertures (2 cm dia.) with doors and baffles, small Al filters | CSL (Centre Spatial de Liège), Belgium, PI: Pierre Rochus | 2 |
METIS/COR | Sun pointing | 2 arcsec over few s | Located behind shield, 1 aperture (17 cm dia.) with door and occulter (8 cm diameter) | INAF (Astronomical Observatory of Turin), Italy, PI: Ester Antonucci | 2 |
STIX | Sun pointing | 2 arcsec over few s | Located behind shield, 1 apertures (12 x 12 cm2) with door (TBC) and filters | Institute of Astronomy, ETH Zürich, Switzerland, PI: Arnold O. Benz | 2 |
SolOHI | Sun pointing |
|
| NRL (Naval Research Laboratory), Washington, D.C., PI: Russell A. Howard | 2 |
In-situ instruments | |||||
SWA | EAS: FOV= 4º sr, | NA | PAS and HIS are S/C body mounted with small (10 cm2) aperture through the heatshield, EAS sensors are on the S/C behind the shield | MSSL (Mullard Space Science Laboratory), UK, PI: Christoper Owen | 4 |
RPW | 3-axis sensing | NA | 3 x antenna on S/C under direct sun light, magnetometer loop and 3 x search coils on the boom | Observatoire de Paris, France, PI: Milan Maksimovic | 5 |
MAG | 3-axis sensing | NA | 2 x sensors located on boom (in the shadow) | ICL (Imperial College London),PI: Tim Horbury | 3 |
EPD | Several directions wrt orbital plane Typical FOV of order 60º x 60º | NA | 5 x sensors located on the spacecraft corners behind the heatshield | University of Alcala, Spain, PI: Javier Rodríguez-Pacheco | 5 |
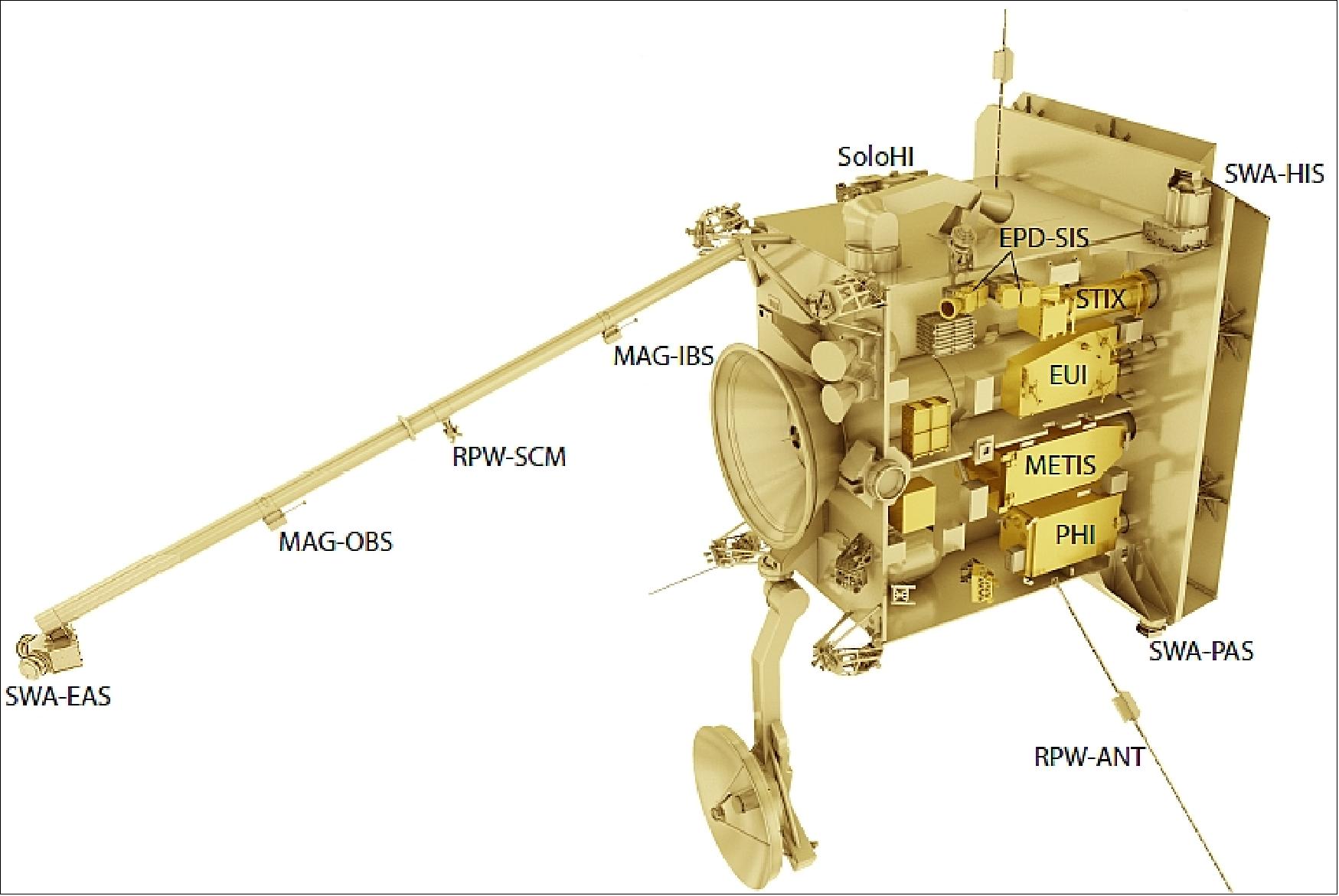
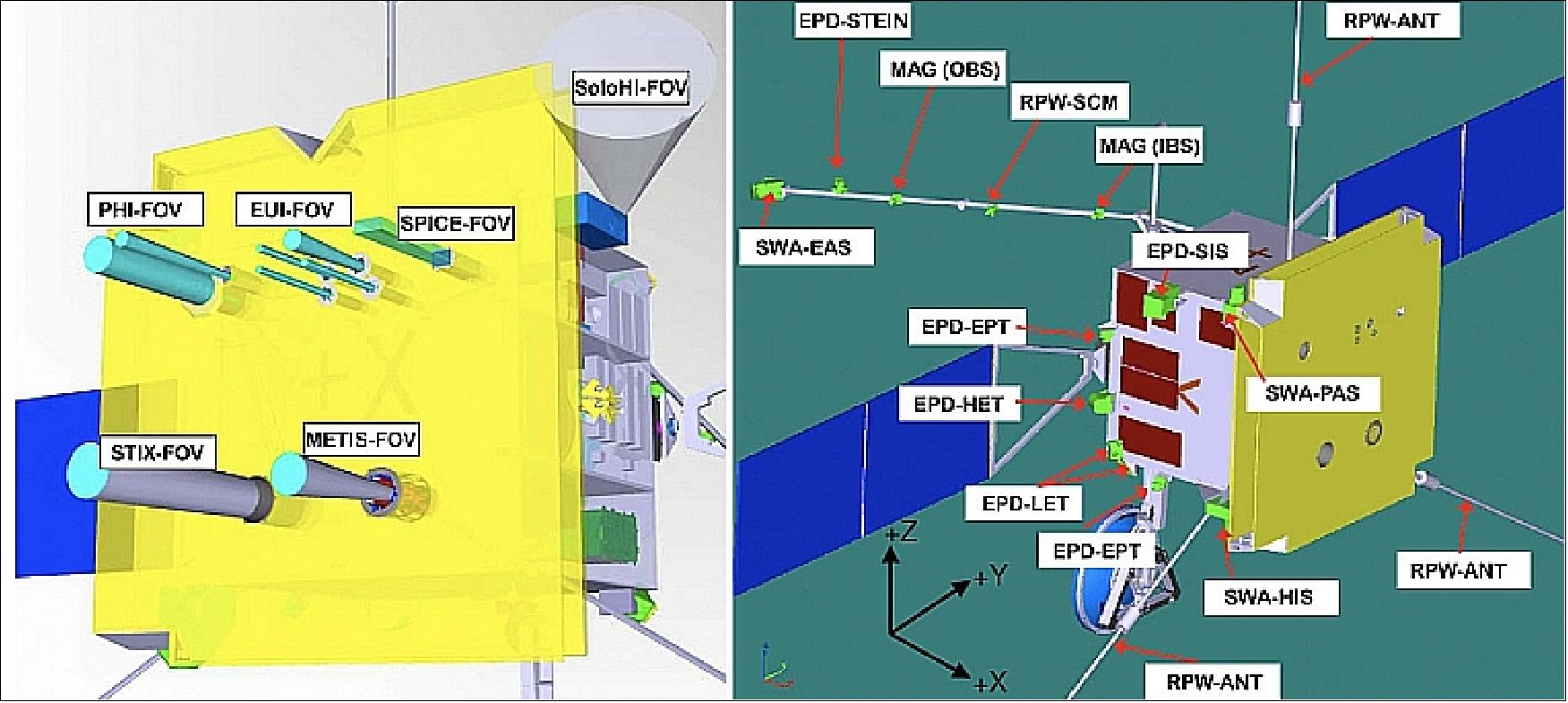
Design Innovations
Although the Solar Orbiter payload is based on heritage from previous solar science missions, the very nature of this mission, along with its constraints, has triggered considerable innovation covering the full range of technical domains (Ref. 68).
ESA spearheaded the investigation of promising new technologies which were considered to hold benefits for the Solar Orbiter payload via several activities in the early phase of the mission (Phase A/B). These included TDAs (Technology Development Activities) for the concept of the PHI HREW (Heat Rejecting Entrance Window), developed under contract with Selex Galileo, and the LCVRs, developed under contract with INTA. Further examples include early UV detector radiation testing and the DRPM (Dynamically Reconfigurable Processing Module) study, which demonstrated the feasibility of the concept of routine partial reconfiguration of the Virtex-4 FPGAs as foreseen in the PHI design.
The instrument teams were involved from the early stages of the requirements specifications for these contracts and were able to follow the progress throughout. Following the end of the activity, responsibility for further development of these items was handed over to the instrument team with reduced risk.
Common procurement: Although the instruments are diverse, wherever possible a common approach to new technologies has been encouraged and facilitated. This is exemplified by the establishment of a CPPA (Central Parts Procurement Agency), which was established by ESA on behalf of the instrument teams for procurement of EEE (Electronic, Electrical and Electromechanical) parts. The service provided to the instrument teams includes the qualification of the EEE components themselves. This provides the opportunity for harmonization and reduces the cost to the instruments. It also lowers the schedule risk associated to qualification at mission level by coordinating procurement milestones and providing visibility to ESA of the qualification progress.
A) Entrance Windows and Filters
Due to the high incident flux, the remote sensing instruments, which require a direct view of the Sun, must reject the majority of the incident energy, while allowing the wavelength range of interest to pass through. This is done in a variety of ways. PHI and STIX have windows located in the spacecraft heat-shield, EUI has entrance filters inside the instrument, while SPICE and the chronographs METIS and SoLOHI have internal mirror systems.
Optical Heat Rejecting Entrance Windows (PHI): Of these the most technologically challenging is the PHI Heat Rejecting Entrance Window (HREW), due to the very narrow requirement for the PHI science wavelength (617.3 nm ± 1.5Å), the large aperture (140 mm for the HRT) and the need to minimize the thermal flux entering the instrument. Although a filtergraph inside the instrument filters the science wavelength to the required level, it is a delicate component which cannot tolerate the full solar load and thus the GREW is the first stage in this filtering process and restricts the incident light to a ~20nm band, allowing only 4% of the total incident energy through.
The concept of the PHI GREW consists of a series of coatings on a glass substrate (Sprawl 300), which filter the wavelength of interest in stages (see Figure 3). In addition to the strict requirements on wavelength pass band, as PHI is an optical polarimeter, there are strict requirements on the polarization, uniformity and wavefront distortion induced by the HREW.
These coatings must be shown to maintain their properties steadily throughout the lifetime of the mission under all operational conditions. This has been validated via a series of environment tests, both at sample and prototype level, involving thermal cycling and radiation tests. A further challenge has been to design a suitable mount for the HREW, which will withstand the vibrational loads while protecting the glass and ensuring that the mount does not disrupt the optical properties of the HREW. The current design has been qualified to 275°C and shown to survive and maintain its optical and thermal performance in all tests, with the exception of a simultaneous radiation test at high temperature in vacuum, which is yet to be performed. In sample level tests, the coated glass has been shown to survive up to a temperature of 350°C without degradation.
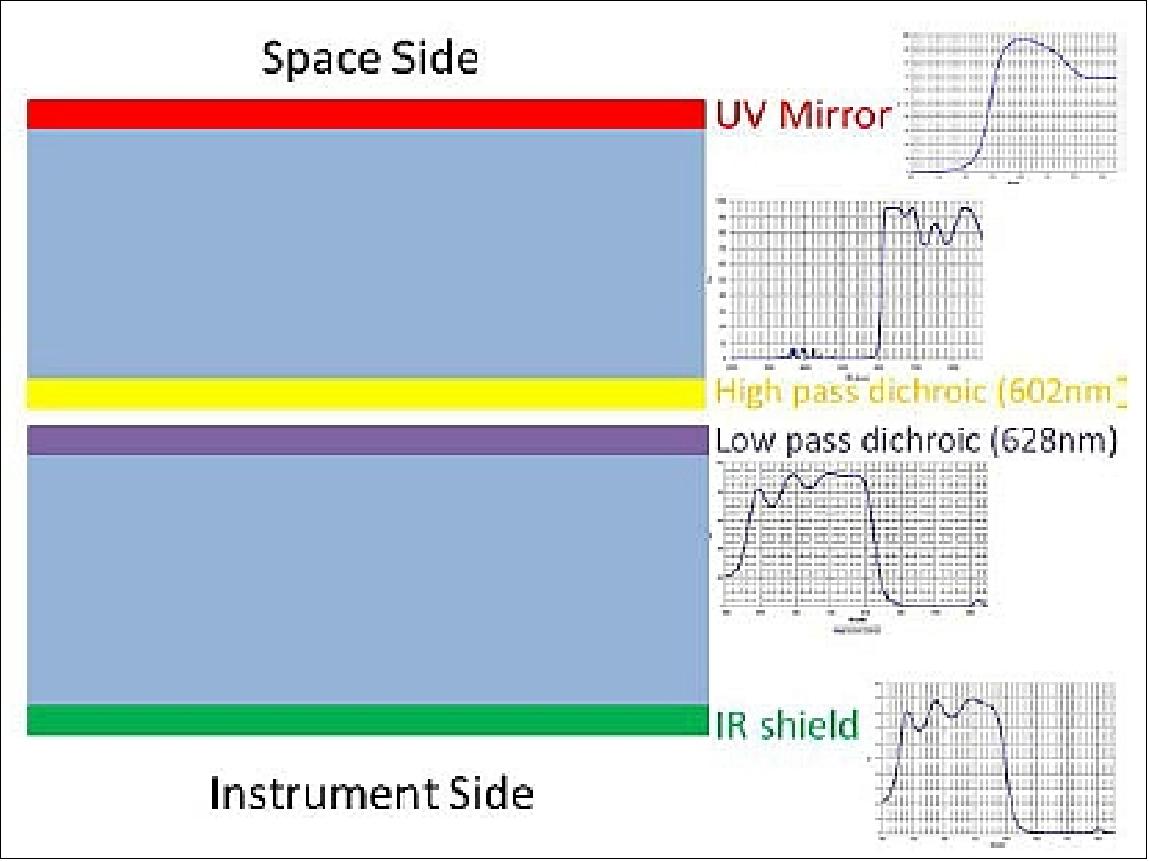
X-ray Windows (STIX): Although it's requirements are not as stringent as those of PHI, STIX needs a window which will be transparent to X-rays (above 4 keV), while minimizing the transmission of lower wavelength ranges (being opaque at wavelengths >300 nm). Due to the nature of the STIX instrument, which involves the generation of Moire shadowgrams on the detectors, the uniformity of the X-ray transmission is crucial, with a requirement of <4% rms variation in the transmission over the aperture (~200 mm diameter). These requirements lead to the need for a Be window with a thickness accuracy of 25 µm. The design involves two windows, one at the front and one at the back of the feed-through in the spacecraft heat-shield.
In order to lower the overall temperature of the window, a protective thermal coating of Al-SiOx is being qualified for use on the sun facing side of each window (Figure 88). For the STIX windows, along with the qualification of the coating, the processes and facilities for manufacture of the window are critical due to the nature of Be dust as a toxic material. Therefore, additional safety precautions are taken in planning and executing qualification tests, such as vibration testing, to ensure sufficient margins of safety against breaking the window.
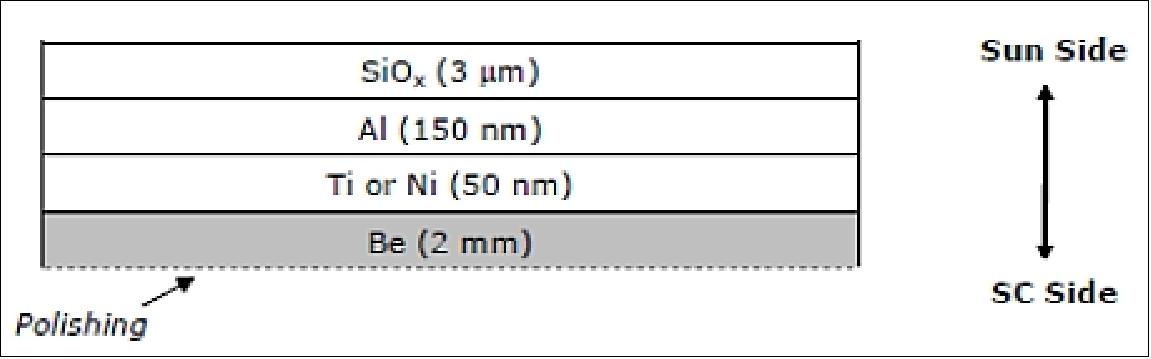
Heat rejecting mirror system (SPICE): The SPICE optics unit (Figure 89) is composed of two parts: the telescope section and the spectrometer section. The primary purpose of the telescope is to reflect and focus as much as possible of the EUV solar radiation in the entire spectral range of SPICE onto the spectrograph entrance slits, while rejecting the unused solar flux (UV/visible/IR).
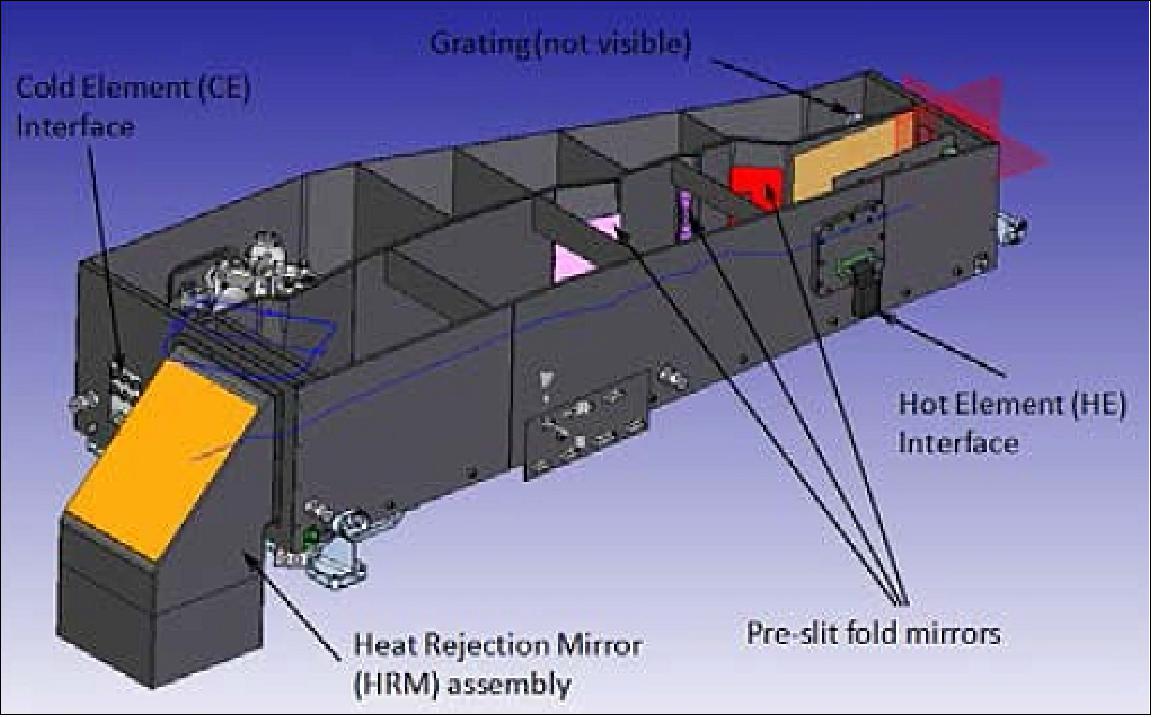
This is accomplished in several steps:
1) The "Solar Transparent" Primary Mirror (PM) is designed with a thin (~10 nm) boron carbide (B4C) coating applied on a fused silica substrate. This provides a VUV (Vacuum Ultraviolet) reflectance of >0.27 between 40 nm and 200 nm. This allows to reflect the EUV radiation of interest for science towards the spectrograph entrance slits.
2) Most of the solar visible and near-infrared radiation is transmitted with little absorption by the PM to the back of the instrument where it is reflected by the HRM (Heat Rejection Mirror) towards outer space. The HRM is a highly reflective vacuum deposited silver fold mirror accommodated inside a CFRP structure ("chimney"). The other internal surfaces of the chimney are uncoated and provide some radiative cooling due to their view to space.
3) The undesired the solar radiation reflected by the PM is reflected to a single heat dump by another set of mirrors in front of the slit. The flux is routed from this heat dump to S/C radiators. These mirrors are configured so that only the required science beam passes through to the slit. Baffles also intercept solar radiation that either diverges as it comes into the instrument or is off-axis due to spacecraft pointing.
Out of the 31.8 W solar load that enters the instrument (end-of-life hot operational case), 22.7 W are rejected by the HRM while only 9.1 W are absorbed internally by the instrument and by the heat dump, as illustrated in Figure 6.
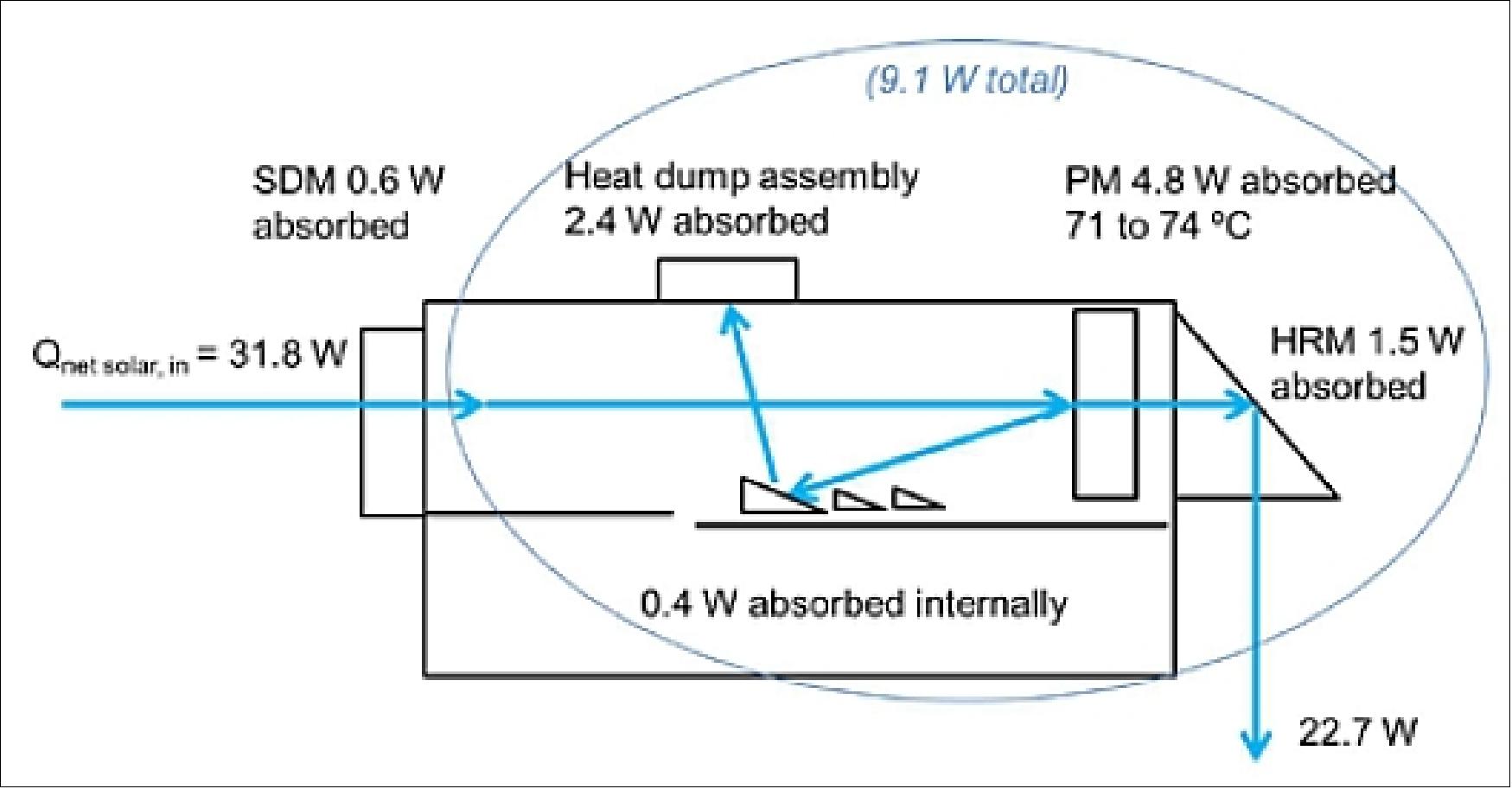
EUV Entrance Filters (EUI): In order to filter the EUV science wavelength of interest for EUI, which is in a narrow passband of 15-31nm, entrance filters for each channel are located at the front of the instrument. These consist of 150 nm thick aluminum filters. The filters receive almost the full bulk of the solar flux (the 47.4 mm High Resolution Imager EUV filter will receive 17.44 kW/m2). While commercial space-qualified filters are available, these have not been qualified to the temperatures and incident solar flux levels which will be experienced by EUI. In addition, due to the very thin nature of these filters, a supporting mesh structure is required to survive the launch loads, however the mesh support in the standard commercial filters do not evacuate the IR load sufficiently.
Developments have therefore been undertaken to customize the filter design, introducing different Ni mesh patterns and also encapsulating the filter in a ribbed frame (Figure 91). Several permutations of the mesh and frame patterns and materials have been trialled to find the optimum combination of transmission performance, thermal conductivity and response to thermal cycling under high temperature and in vacuum. CSL have also investigated a prototype in which the supporting grid and frame are grown simultaneously on the Al filter ("grid-on-filter"). These investigations have led to a convergence on a custom design involving a spiral Al frame/rib pattern matching that of the underlying Ni mesh (Figure 91). This has been tested successfully to 13 SC.
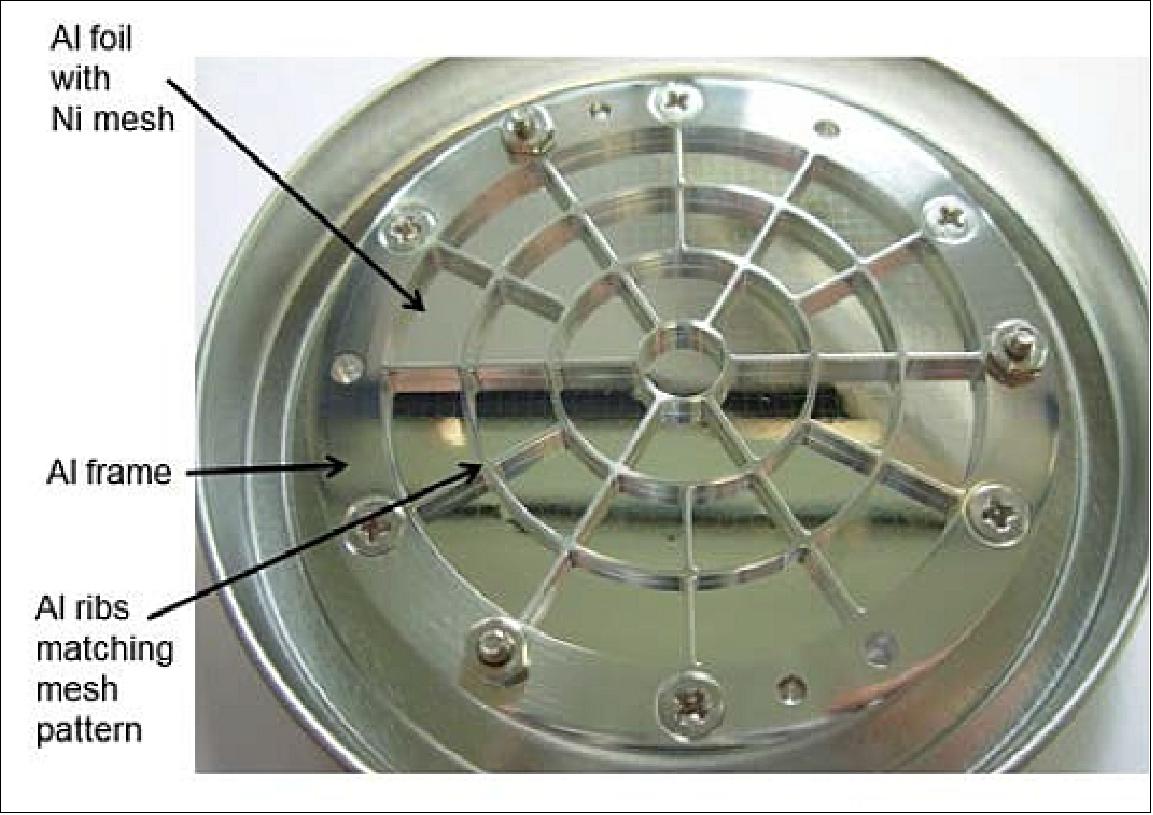
B) Polarizers and Other Optics
LCVR (Liquid Crystal Variable Retarder) technology introduction, (PHI and METIS): The polarization measurements in the PHI and METIS instruments are performed by means of LCVRs, a technology which has considerable heritage in ground- and balloon-borne instruments but has not yet flown in space. These polarizers consist of LC (Liquid Crystal) cells, which are electrooptical polarization modulators. By adjusting the voltage applied to the cells, the orientation of the LC molecules is changed and this changes the optical retardance (Figure 92). In the case of PHI, two LCVR cells will be oriented with their fast axes at 45° to each other as part of the PMP (Polarization Modulator Package), to enable differential retardance of the different polarization states. 70)
The advantages of this technology for space applications derive from the low resource demands (power, mass and volume) as well as the fact that it avoids the use of mechanisms and is easy to control and to synchronize with the detector readout.
The qualification activities performed for these cells included the investigation of several material types as well as the design of a mount assembly. The optimized design successfully passed mechanical, thermal and radiation tests such that TRL (Technology Readiness Level) 5 was reached in mid-2011.
The PMP for PHI consists of two APAN (Anti-Parallel Nematic) LCVRs oriented with their fast axes at 45° with respect to each other followed by a linear polarizer (the polarizing beam-splitter, the polarization analyzer) aligned with the fast axis of the first LCVR. The PMP generates four modulations of the polarization state in order to extract the Stokes parameters of the solar incoming light.
For METIS, only one LCVR is necessary in the PMP with a quarter-waveplate (not included in the PMP, but placed previously) in order to analyze the linear polarization. Nevertheless, an additional LCVR has been included with its fast axis parallel to the first cell, but with the pretilt angles of the liquid crystal molecules in opposite direction to obtain an extended and wider acceptance angle.
Obviously, in both instruments, a linear polarizer is included before the detector to be able to analyze the signal as change in the detected intensity.
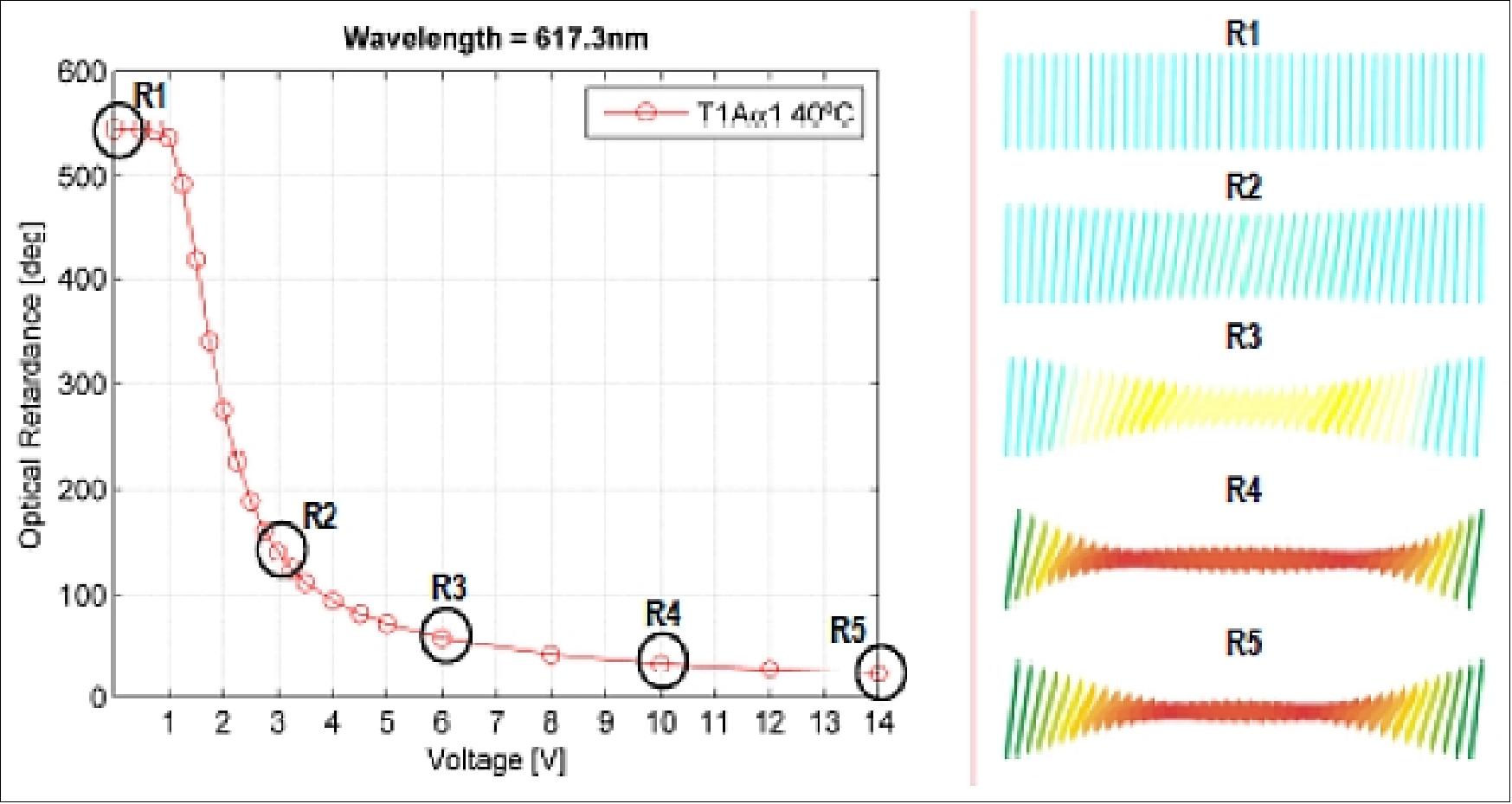
Etalon (PHI): The fine filtering of the PHI science wavelength to the required narrow passband (optimum bandwidth in the range 80-120 mÅ) is performed in a Filtergraph consisting of Lithium Niobate (LiNbO3) electro-optic etalon. This is a single crystal with a clear aperture of 50 mm and fabrication finesse of ≥ 30. The etalon needs to be tuned in order to scan the spectral line as well as to compensate for the spacecraft radial velocity and this is performed by adjusting an applied high voltage. The temperature sensitivity of such an etalon is 2.9 pm/K, which means that the etalon must be kept in a temperature controlled oven, set to a temperature which can be maintained throughout the operational orbit.
As in the case of the LCVRs, the etalon wafers are commercially available and have been used on balloon-borne instruments, however they have not as yet flown on a space mission. A qualification for the Solar Orbiter is being undertaken, of which vibration testing, vacuum testing and proton irradiation testing up to 60 krad on a structurally representative sample have so far been performed successfully.
C) Detectors and Electronics
Back-illuminated CMOS detectors: The detectors for each of the three channels of the EUI (Extreme UV Imager) instrument (Table 4) are based on CMOS APS technology. Prototype 1 k x 1 k front-thinned, double-gain detectors (APSOLUTE) have been developed for EUI, in parallel to similar 2 k x 2 k single-gain prototype development for PHI. These have successfully undergone testing to demonstrate suitability for the Solar Orbiter environment.
However further improvements on these designs are needed in order to fulfil the EUI science objectives:
- While front-thinned detectors are suitable for the EUI Lyman-α channel, the EUV channels require a new development of back-thinned detectors. This is needed in order to allow back-illumination of the detectors to achieve the required sensitivity in the EUV 10-40 nm range (Figure 93)
- Double gain, as developed in the APSOLUTE prototypes is needed in order to minimize the read-out noise and increase the speed and dynamic range.
- For the Full Sun Imager, the array size has to be increased to 3 k x 3 k in order to achieve the required FOV and angular resolution.
Each of these new developments presents its own challenges and full qualification of the new design for Solar Orbiter must be performed. This activity is currently underway.
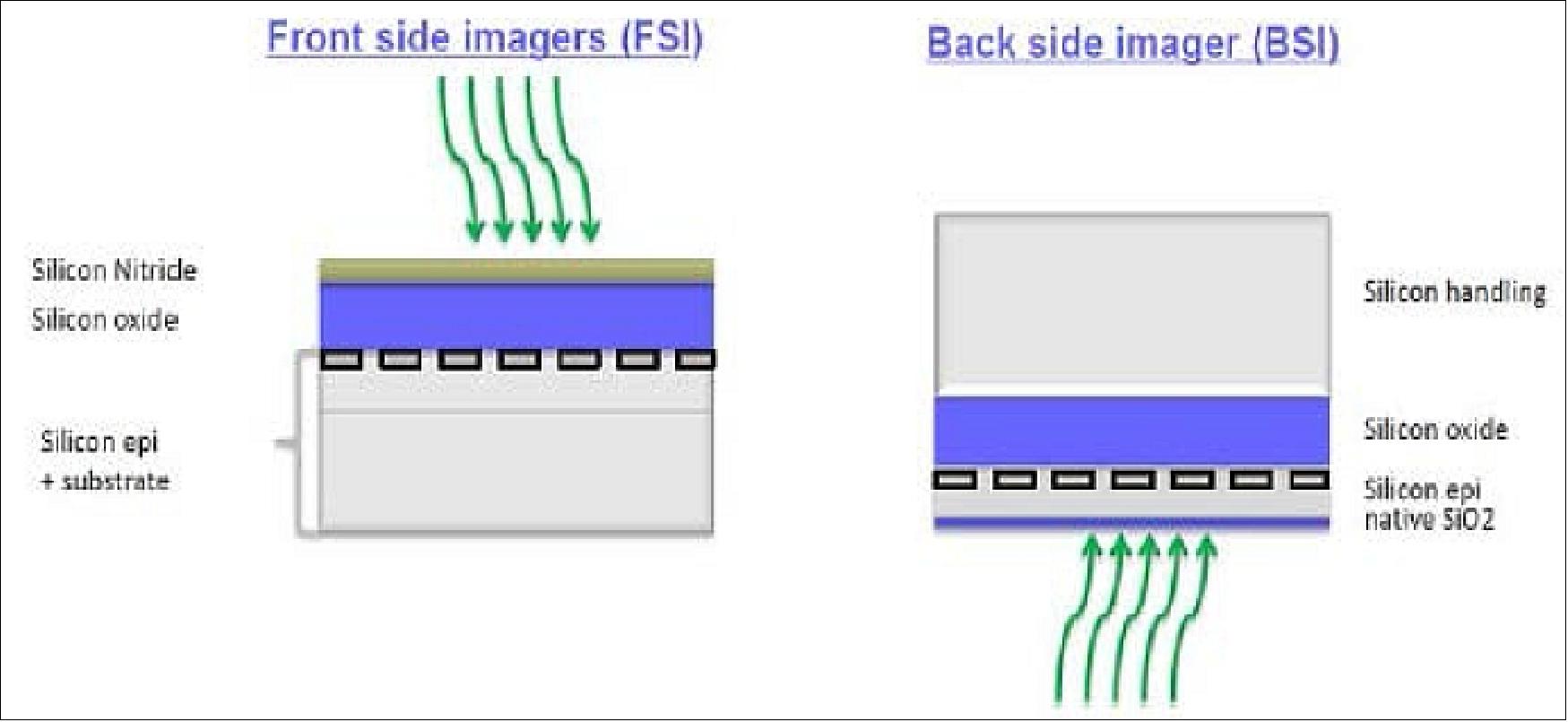
IDeF-X ASIC (STIX and EPD): The IDeF-X (Imaging Detector Front-end) ASIC is a new development in Front End Electronics which may be flown for the first time on Solar Orbiter in the STIX and EPD (Energetic Particle Detector) instruments. STIX will use the IDeF-X HD (High-Dynamic) version, while the IDeF-X BD (Bi-directional) version will be used in EPD-STEP.
For STIX, the ASIC is integrated in the Caliste-SO detectors, which are hybrid components integrating a pixelated 10 mm x 10 mm CdTe sensor with its HV power supply, the analog front end read-out electronics in the form of the IDeF-X HD, passive components and a 20 pin surface mount interface (SOP interface). The µPCBs are stacked perpendicular to the CdTe sensor in the Caliste-SO body (Figure 94).
The IDef-X performs the read-out of up to 32 channels in terms of pixel location, energy and rise time. The design involves a CSA (Charge Sensitive Amplifier) stage followed by a pulse shaper, with several tunable parameters, including CSA bias, peaking time and low level discriminator. Different (partial) channel read-out modes can be configured in order to optimize power consumption.
The advantages of this ASIC are primarily in terms of savings on volume, mass and power. The power consumption per channel is 800 µW. As the detectors have to be cooled in order to minimize the noise and leakage current, savings in power consumption and thus heat dissipation are very beneficial.
The characterization of the Caliste-SO prototype has shown its ability to meet the STIX performance requirements and full qualification of this device is underway. 71)
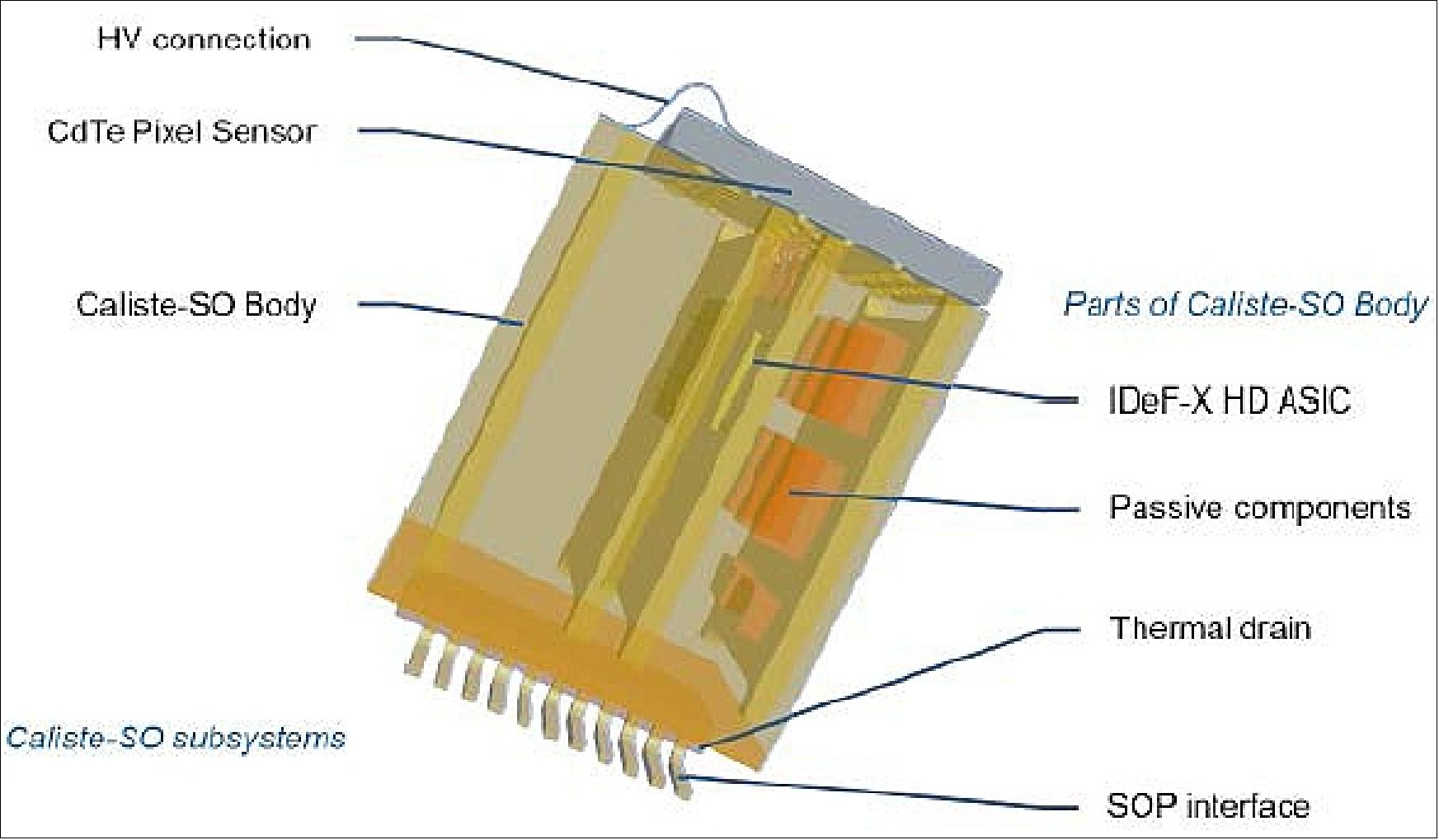
Virtex-4 FPGA (PHI): The full data processing chain of PHI requires to perform an inversion of the RTE (Radiative Transfer Equation) to derive the magnetic vectors and line of sight velocity. Due to the limited telemetry bandwidth on Solar Orbiter, this must be performed onboard as there is insufficient bandwidth to downlink the raw PHI data. Such onboard processing can only be performed by an FPGA such as the Xilinx Virtex-4, while remaining within the instrument power budget. PHI are thus planning to use two Virtex-4 CF1140 devices (1140 pins) in their DPU (Data Processing Unit).
While the Virtex-4 component itself has been qualified for use on space missions, there is currently no assembly house in Europe which is space-qualified for soldering the CF1140 package of this component. Thus, the PHI project is undertaking a dedicated qualification program for the assembly of this device, involving the manufacture of dedicated verification boards which will undergo vibration and thermal cycling tests to simulate the mechanical strain to the solder joints which may be experienced during the mission.
D) On-board Data Processing
Routine FPGA reconfiguration (PHI): In addition to the processing required for the RTE inversion, PHI require considerable on-board processing capability for instrument functionality such as control of the Image Stabilization System and data pre-processing.
To optimize mass, volume and power, the PHI project has developed a design making use of the ability to re-program the Virtex-4 FPGAs to routinely switch the FPGA code in order to perform different functions at different points in the orbit, as follows (Figure 95):
- In one configuration, FPGA1 performs ISS (Image Stabilization System) control and FPGA2 performs the data accumulation.
- In the second configuration, FPGA1 performs the RTE inversion and FPGA2 performs the data pre-processing.
While reprogrammable FPGA technology has been flown in space on several missions, the PHI architecture involving routine changes of the FPGA code throughout the mission is novel and relies on the robustness of not only of the FPGA code protection but also that of the SoCWire network between the components. This is also under verification as part of the ESA DRPM (Dynamically Reconfigurable Processing Module) study.
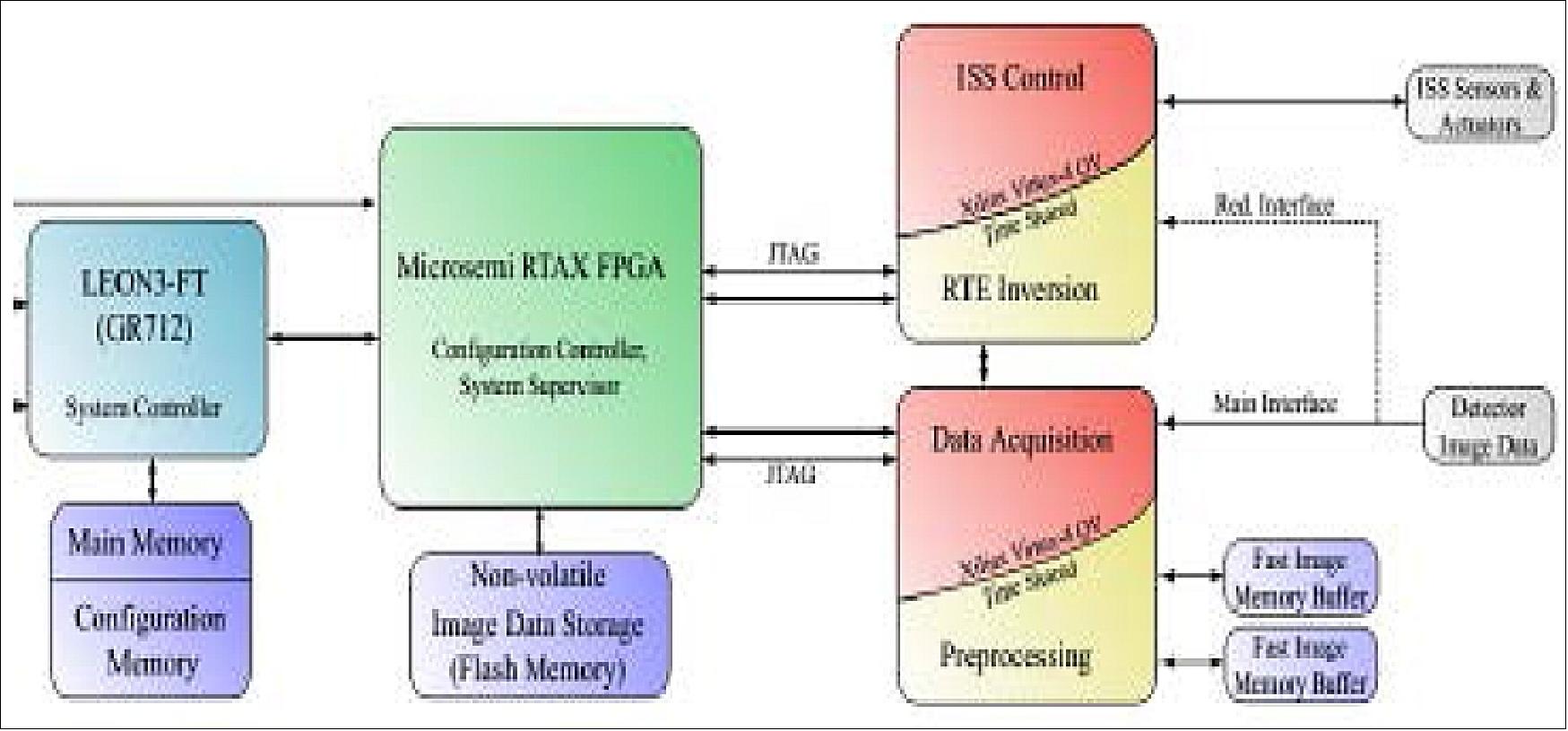
E) Deployment Mechanisms
Antenna deployment mechanism (RPW): The RPW (Radio and Plasma Wave) antenna (ANT) system consists of three identical antennas which are oriented at angles of roughly 120º in the operational configuration. Each antenna comprises a 5 m long sensor and an almost 1 m long boom that keeps the sensor at a distance from the spacecraft to reduce disturbances and supports the preamplifier close to the sensor. Due to the antenna length it is obvious that the antennas need to be launched in stowed configuration and deployed in orbit. As most of the length of the antennas sticks out from the heat shield, material selection must take into account direct sun illumination.
The general antenna design is based on several pipe segments connected by "Maeva" elastic hinges, the deployment of which is controlled by SMA (Shape Memory Alloy) components. In stowed configuration each antenna is fixed by three HDRMs (Hold Down and Release Mechanisms) which are opened in sequence to release the antenna. For each HDRM, two stowing flanges are guided by journal bearings and driven to their open position by springs, once a Frangibolt non-pyrotechnical device (SMA based) is actuated. The stowed antenna assemblies are protected by MLI shells in order to keep the temperature of the SMAs in the Frangibolts and hinges below the transformation temperature, thus avoiding premature and uncontrolled deployment. The MLI shells are mounted on carbon tubes that are attached to the HRDM flanges.
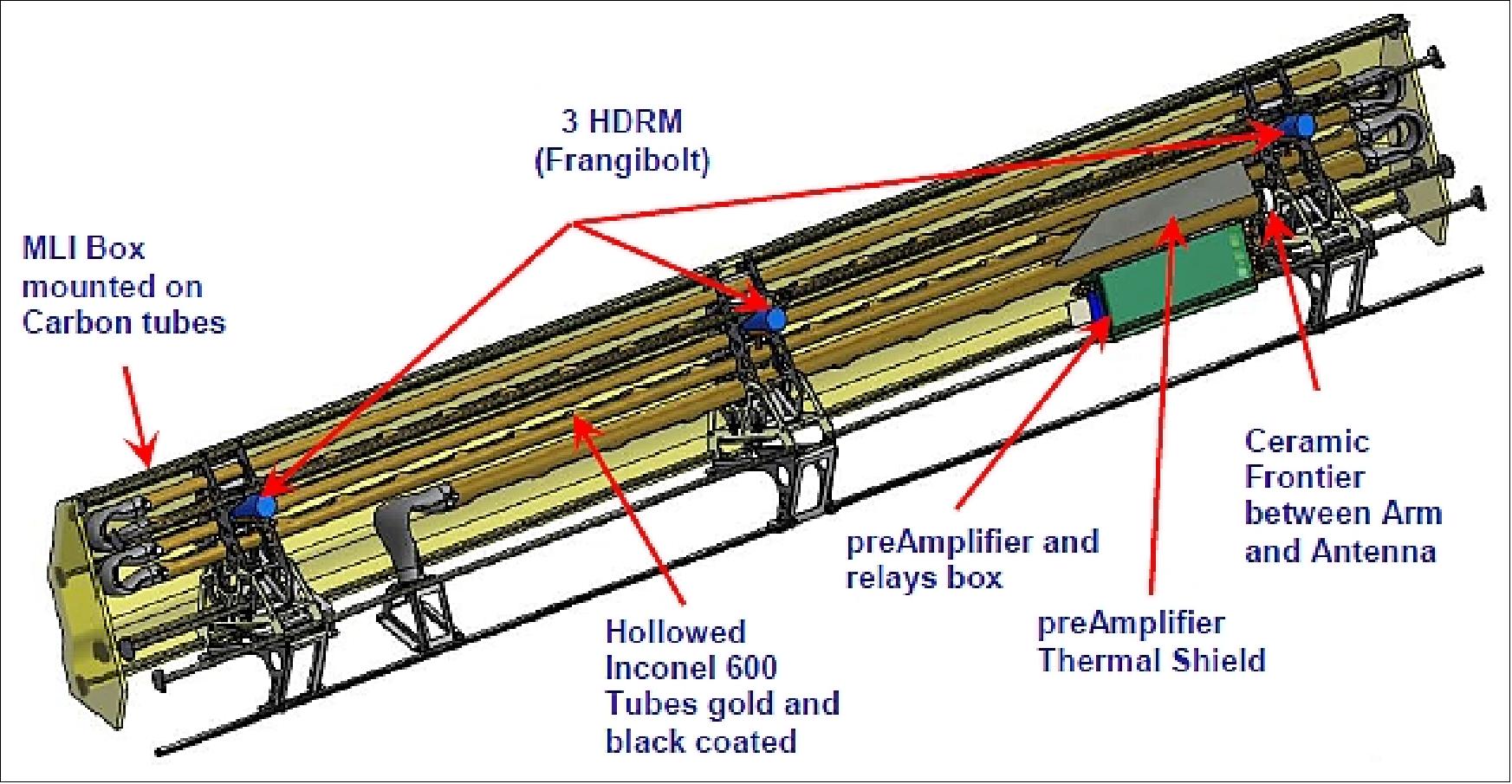
The Maeva hinges, controlled by the SMA components, were developed by CNES. The Maeva hinges constitute Carpentier elastic blades that ensure both deployment and locking of the hinges. To control the deployment, each hinge is equipped with two SMA strings around which Cerafil heater wires are wound. For each antenna, all SMA heaters are electrically connected in series.
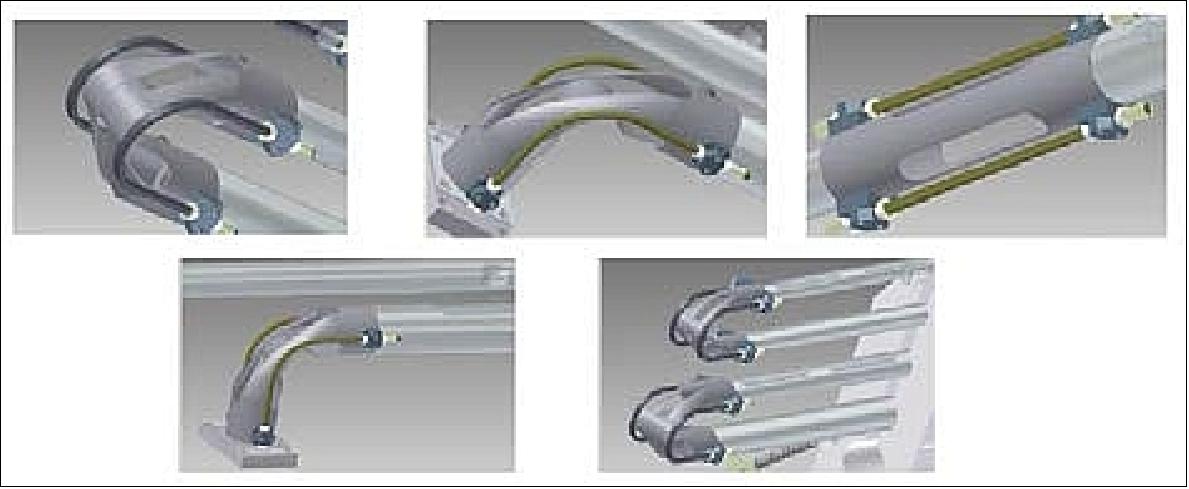
Mechanical, thermal and functional (in particular deployment) tests are being performed on full antenna models and subsets. High temperature materials are also being qualified separately.
Solar Remote-sensing Instrument Package
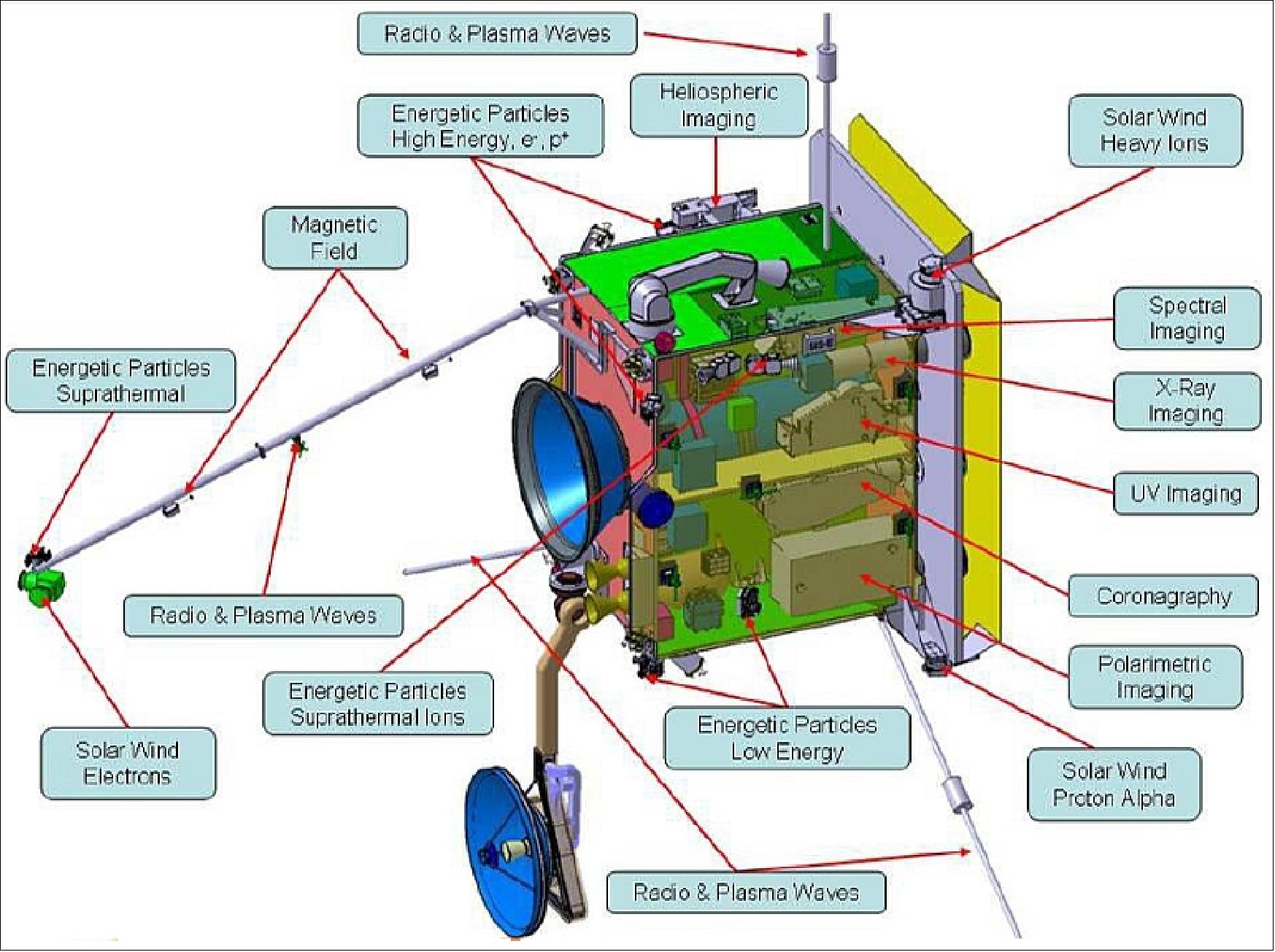
PHI (Polarimetric and Helioseismic Imager)
The PHI instrument is a diffraction limited, wavelength tunable, quasi-monochromatic, polarization sensitive imager which is being developed by a consortium with major contributions from institutes in Germany, Spain and France, and smaller contributions from Sweden, Norway and Switzerland.
The objective of PHI is to measure the magnetic vector and the LOS (Line-of-Sight) velocity fields in the solar photosphere. It will thus probe the deepest layers of the Sun (including the solar interior using helioseismology) of all the instruments on Solar Orbiter or the Inner Heliospheric Sentinels. Since the magnetic field anchored at the solar surface produces most of the structures and energetic events in the upper solar atmosphere and significantly influences the heliosphere, PHI plays a key role in reaching the science goals of Solar Orbiter. Extrapolations of the magnetic field observed by PHI into the Sun's upper atmosphere and heliosphere will provide the information needed for other optical and in-situ instruments to analyze and understand the data recorded by them in a proper physical context. 73) 74) 75)
The instrument concept uses two telescopes:
• HRT (High Resolution Telescope) with an aperture diameter of 125-160 mm which achieves an angular resolution of 1.00 arcsec (150 km on the Sun at minimum perihelion distance of 0.22 AU). Its FOV (Field of View) will be 1000 x 1000 arcsec. The off-axis Ritchey-Chrétien HRT will image a fraction of the solar disk at a resolution reaching 150 km at perihelion (the same resolution as the Extreme Ultraviolet Imager's high resolution channels will have).
• FDT (Full Disk Telescope) with an aperture diameter of 15 mm providing an image of 2k x 2k pixels. The refractor FDT will be able to image the full solar disk at all phases of the orbit. It incorporates an off-pointing capability. 76) 77)
Each telescope will have its own PMP (Polarization Modulation Package) located early in the optical path in order to minimize polarization cross-talk effects. Polarimetry at a signal to noise level of 103 is baselined for PHI. The HRT and the FDT will sequentially send light to a Fabry-Perot filtergraph system (~ 100 mÅ spectral resolution) and on to a 2048 x 2048 pixel CMOS detector. PHI will have its own ISS (Image Stabilization System ) that will compensate spacecraft jitter or other disturbances. This system will be composed of a limb sensor and separate rapid tip-tilt mirrors for the FDT and the HRT.
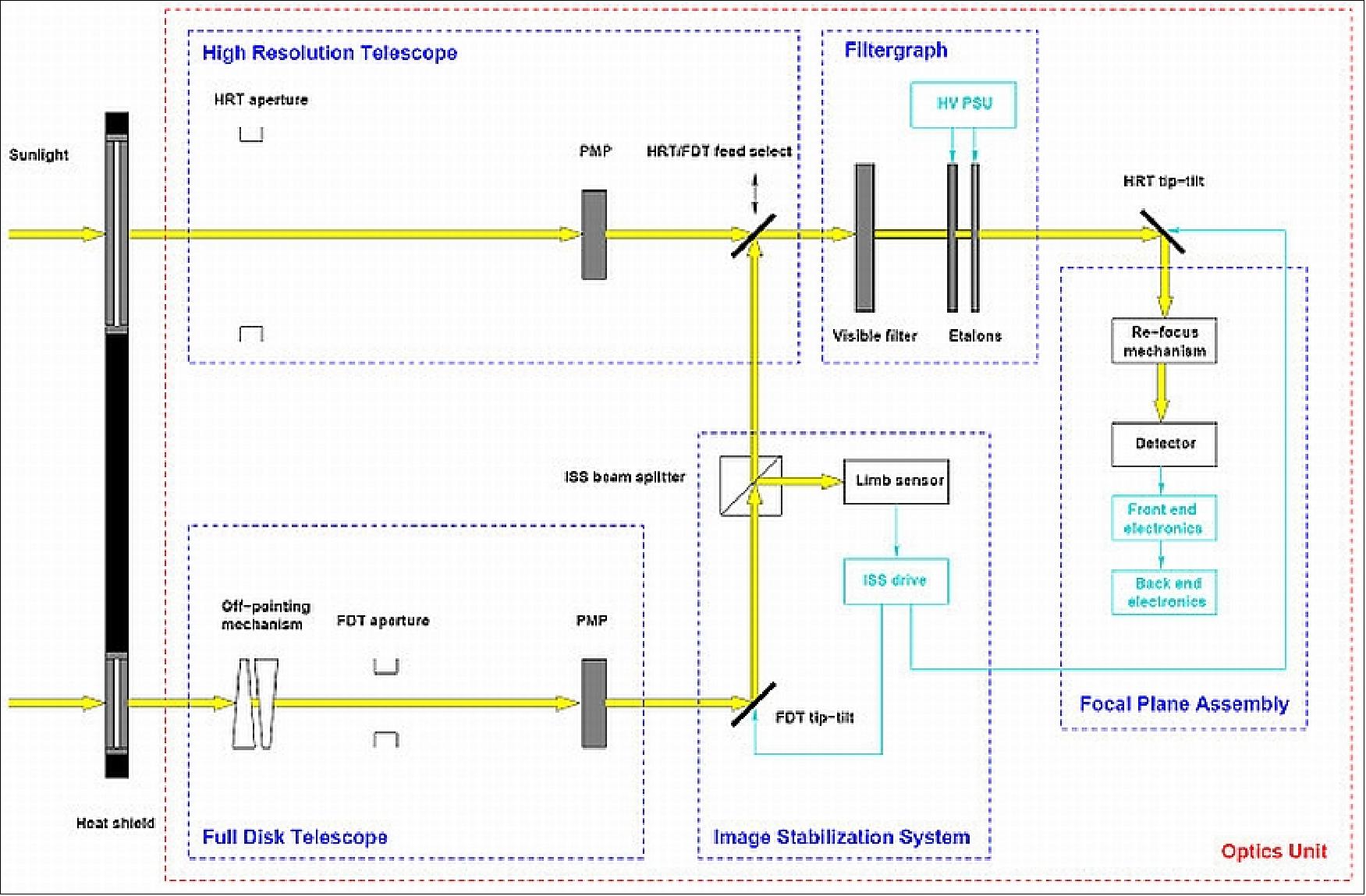
MPS (Max Planck Institute for Solar System Research) of Lindau, Germany has system responsibility for the implementation of the PHI instrument. This comprises the development of a stable housing and its connections to the spacecraft's main body. Due to the close solar proximity during Solar Orbiter's perihelion passages, all instrument components are subject to stringent conditions regarding radiation hardness and thermal stability.
The MPS is responsible for the full optomechanical development of the HRT (High Resolution Telescope). The prospective optical concept is based on an off-axis Ritchey-Crètien telescope with an aperture diameter of 160 mm and an effective focal length of approximately 2500 mm. An off-axis design is preferred since it causes reduced thermal stabilization complexities which pose the main challenges of the instrumental design. In addition, a feed select mechanism for choosing between the two telescopes will be developed under authority of the MPS. In addition, MPS provides the focal plane assembly of the PHI instrument. This comprises the development of a fast 2 k x 2 k APS-detector system and the associated front-end electronics which can be qualified for the space mission.
The Spanish institute IAC (Instituto de Astrofísica de Canarias) is a partner of MPS. The consortium includes several other Spanish and German laboratories and the CNRS/IAS (Institut d'Astrophysique Spatiale) of France.
PHI will allow high-resolution and full-disk measurements of the photospheric vector magnetic field and line-of-sight velocity, as well as the continuum intensity in the visible wavelength range. For these objectives the instrument will consist of two telescopes, one to image a fraction of the solar disk at high spatial resolution and the second to image the full solar disk at all phases of the orbit. Both telescopes will be used sequentially to carry out narrowband measurements. Figure 100 shows the Solar Orbiter orbit with the radial velocity (i.e. relative to the Sun) as a function of the heliocentric distance. 78)
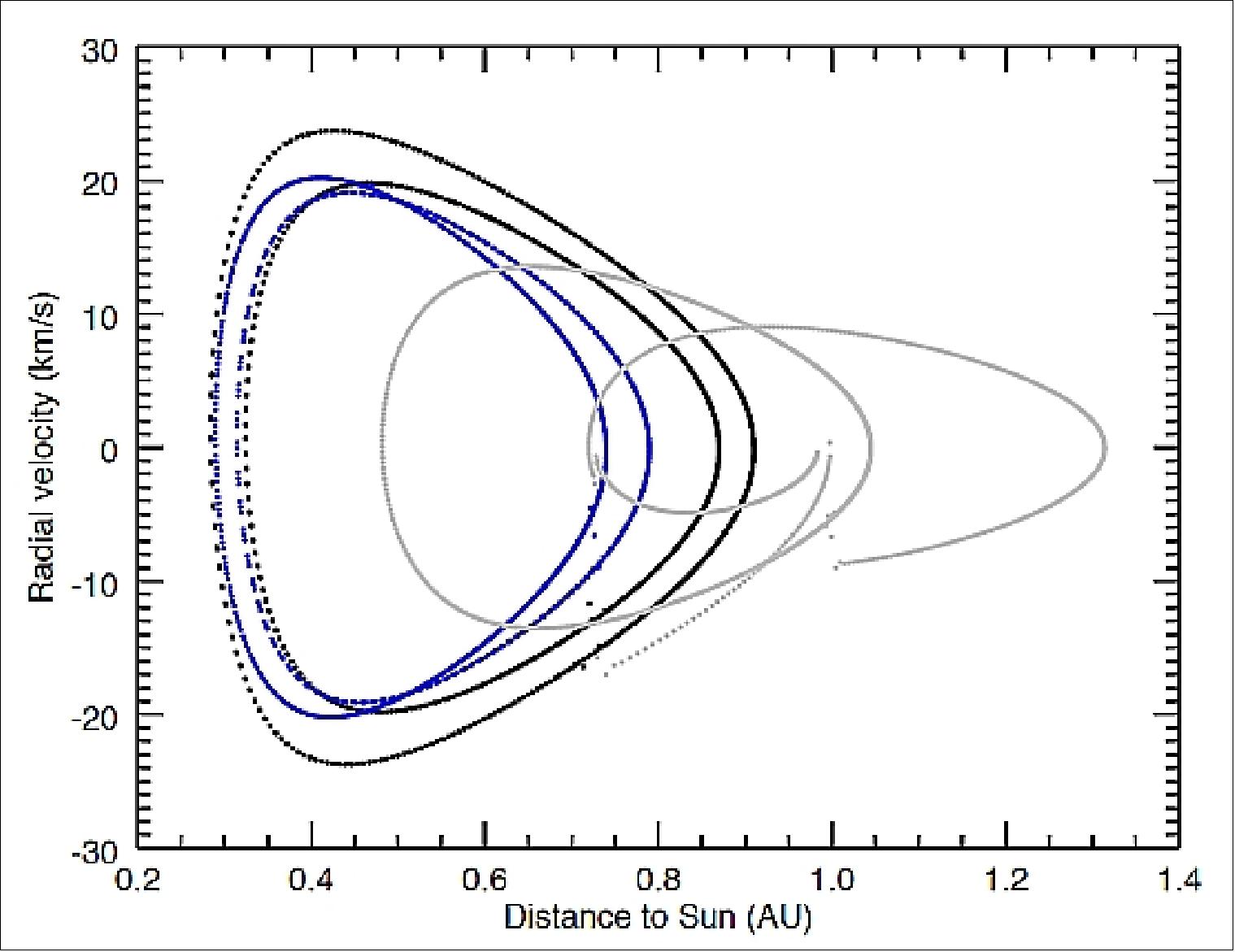
FG (Filtergraph): PHI will carry out the measurements observing the Doppler- and Zeeman-effects in the FeI 6173 Å absorption line (sensitive to magnetic field). This line will be scanned with a narrowband FG. At different spectral positions, the polarization state of the incoming light will be analyzed. The FG will provide a tuning range of ±0.6 Å to follow the wavelength shift produced by the spacecraft radial velocity (i.e. up to 26 km/s along the orbit), plus the range required to scan the absorption line and the continuum. It will be tuned at six wavelength positions plus one for the continuum (Ref. 78).
The FG has been designed by IAS using the optical design provided by MPS. The design is based on previous experiences and includes a solid Fabry-Perot etalon and a narrowband prefilter. It extracts a spectral portion of the FeI 6173 Å absorption line and a nearby continuum point. To achieve this objective, it will select a passband of 0.1 Å. The etalon is made of Lithium Niobate (LiNbO3). This type of etalon is smaller and lighter than the piezo-stabilized ones. The temperature stabilization is very important since the tuning of the etalon can be lost as a consequence of temperature variations. Given that the performance of the etalon is optimum at normal incidence, the FG has a telecentric optical design. A prefilter blocks the secondary transmission peaks from the etalon. It is formed by two components: PF1 and PF2. The first is the narrow passband and the second one is the blocker for all the spectral range. The combination of both filters achieves the maximum transmission inside the passband and the maximum out-of-band rejection. PF1 has a passband width less than 3 Å. It is very sensitive to the temperature and to the AOI (Angle of Incidence).
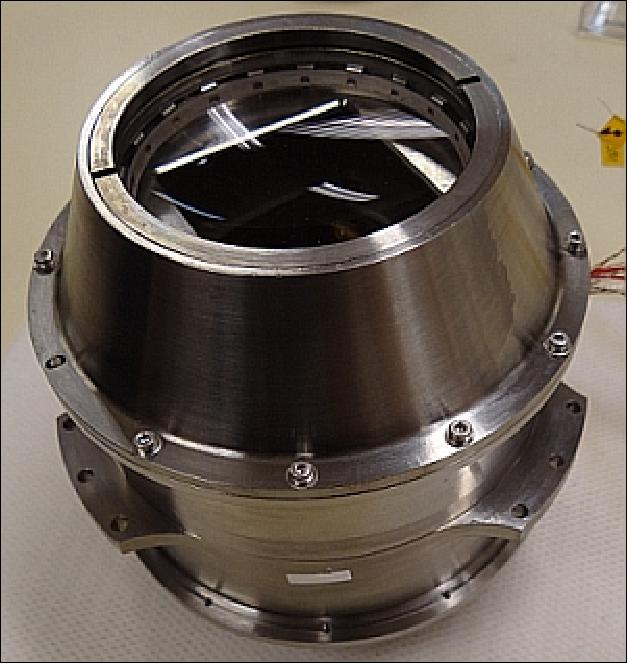
As it can be seen from Figure 100, the heliocentric distance and the radial velocity will vary significantly along the observations. The distance to the Sun will go from 0.28 AU to 0.9 AU and will have a direct effect on the instrument temperature, therefore the FG is designed to keep the etalon and prefilter at a constant temperature. Figure 101 shows the picture of the FG prototype completely assembled. All the components, with the exception of the lenses are placed inside a thermally-controlled oven.
The fine tuning of the FG is essential for the instrument scientific exploitation. The tuning will rely on the ground characterization and on the on-board calibration. Both constitute an important milestone in the development of the instrument. The ground characterization is currently being performed at IAS.
The FG characterization includes optical, electrical and mechanical tests. Its objective is to assess the spectral response, the image quality, the stability and the operation life. It will be used to build the look-up tables included in the on-board calibration program. These tables are the optical transmission maps as a function of wavelength and the spectral response as a function of the tuning voltage. As summarized in Table 5, each optical component is being tested separately and, once integrated in the FG as a complete system. The tests include measuring the transmission profile all along the useful surface, the AOI sensitivity, the thermal gradients across the useful surface, the tuning stability and the thermal stability. These measurements have been ranged according to their priority and complexity. They are done in air and in vacuum conditions. A special setup has been prepared for the tests in a 10000-class clean room and in a solar telescope (i.e. the Tour Solaire at Meudon, France).
Component | Test |
PF1 (Passband Filter 1) | Transmission profile, thermal gradients, AOI (Angle of Incidence) and temperature sensitivities |
PF2 (Passband Filter 2) | Transmission profile |
Etalon | Transmission profile, thermal gradients, AOI, temperature and high voltage sensitivities |
Assembled FG (Filtergraph) | Transmission profile, thermal gradients, alignment, thermo-elastic stability and operation life |
The test setup for these tests includes one "in-air" chamber and three vacuum chambers of different sizes, all of them with temperature control. Different light sources are in use: Sun light, monochromatic and white light. One CCD and two calibrated photodiodes are used for the visible and the near-infrared measurements. In addition, the test setup comprises several high-precision mechanisms to align and tilt the components. The spectrometers in the test setup have a resolving power (R) of 15 x 103 for the broadband measurements and 3 x 105 for the narrowband ones.
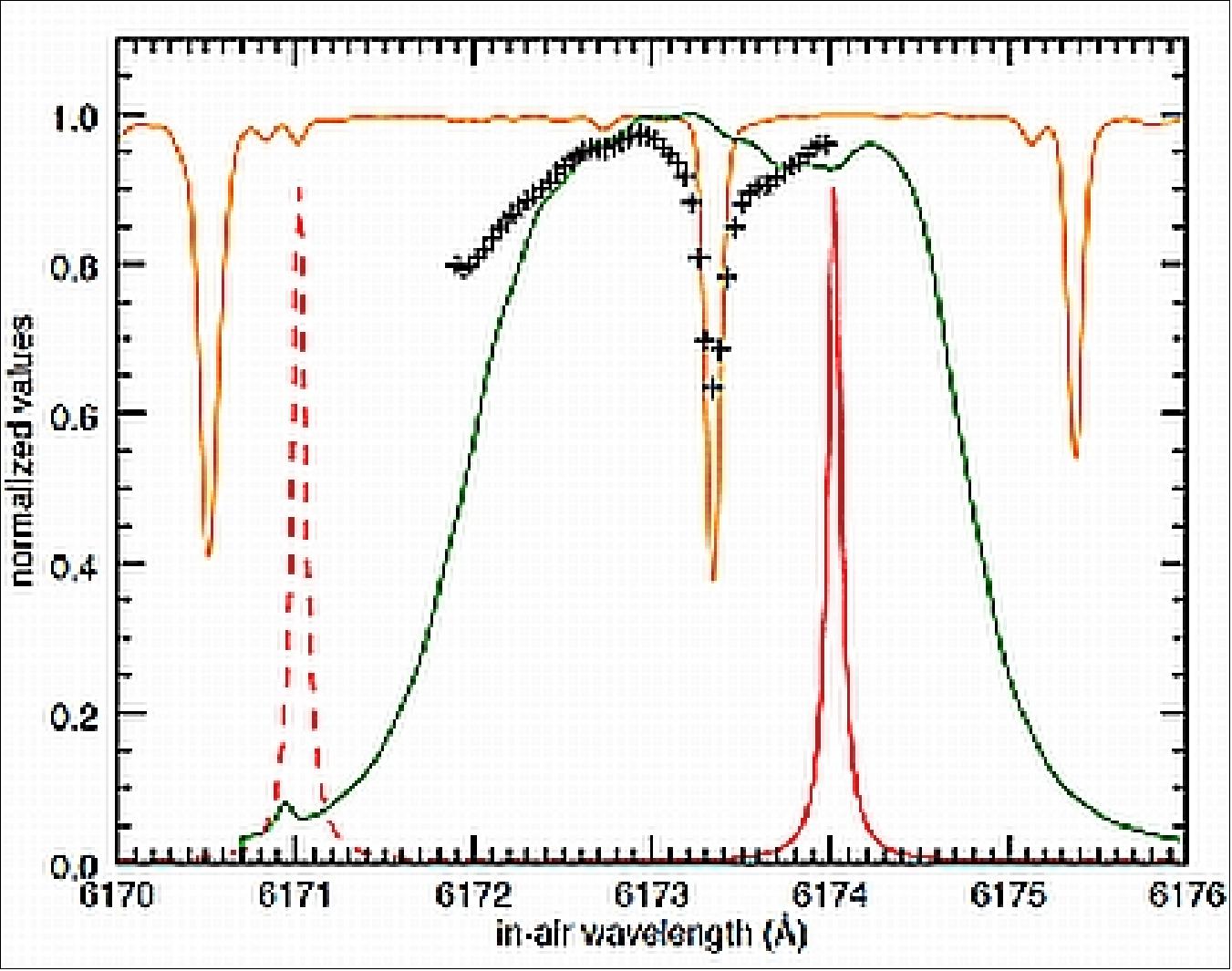
Figure 102 shows the measured PF1 narrowband transmission and the simulation of the spectral scan that will be obtained by the FG. It is the result from the convolution of the solar spectrum with the prefilter measurement and with the etalon profile being tuned step by step in the wavelength range shown. Figure 102 shows the dependency on the prefilter transmission profile, thus the importance to measure it with high precision. Currently, the prototype of the prefilter has been measured to 0.06% (1σ error) with the spectroscopic test setup at the solar telescope.
The PHI instrument has a mass of 34 kg, power consumption of ~31 W, and a date rate of 20 kbit/s.
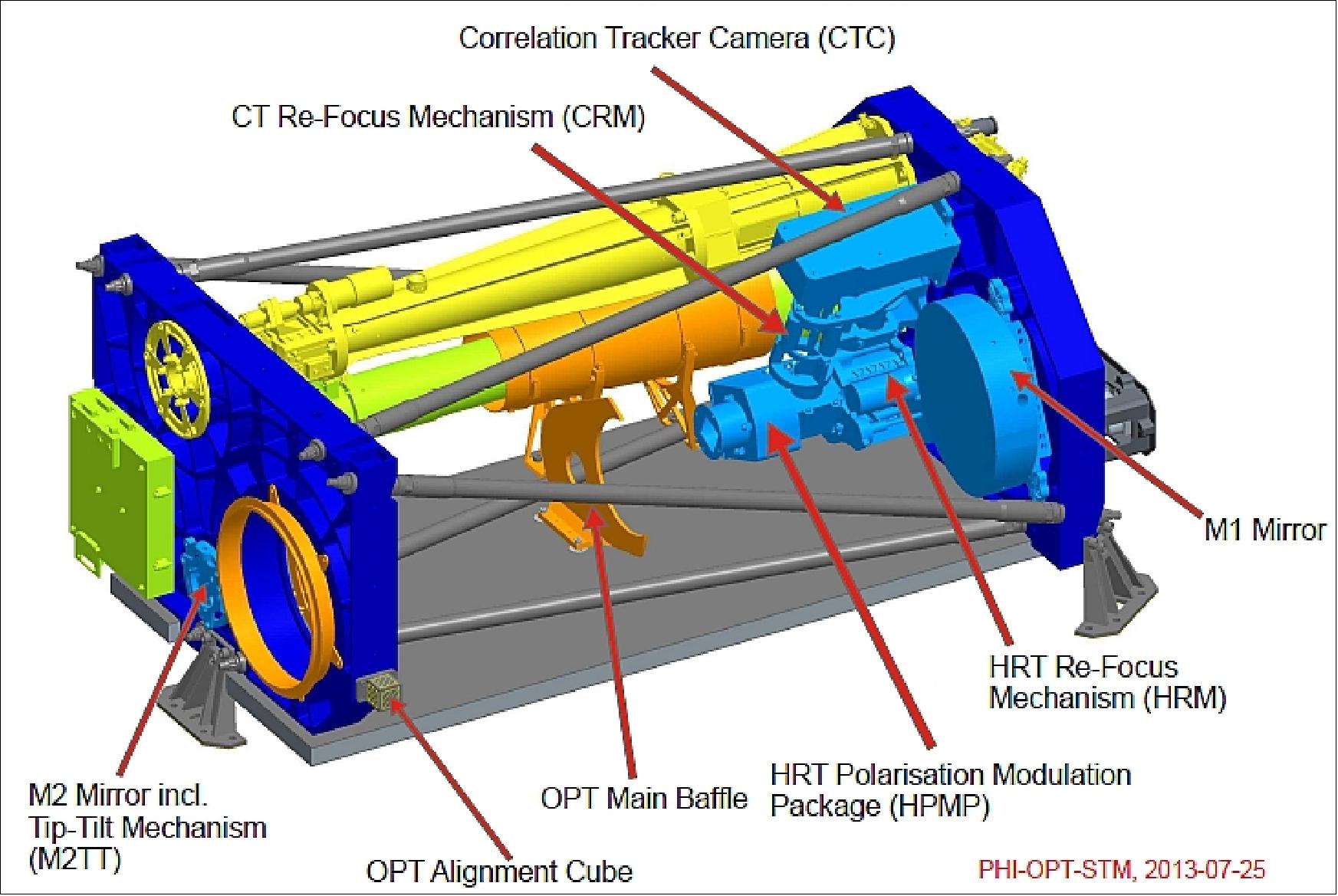
SPICE (Spectral Imaging of the Coronal Environment)
SPICE is an EUS (Extreme-Ultraviolet Spectrograph) imager for the Solar Orbiter mission. The SPICE instrument was selected as a NASA contribution to SolO mission under the PI-ship of SwRI (Southwest Research Institute). This instrument is designed to make observations in the far and extreme ultraviolet and will provide plasma diagnostics of the solar atmosphere where the temperature ranges from tens of thousands to several million degrees.
MPS (Max Planck Institute for Solar System Research) of Lindau, Germany is actively participating in the design of the SPICE instrument. The main MPS contribution is the design and development of the primary telescope mirror. The primary mirror plays a key role in the optical and thermal design of the SPICE spectrograph. The off-axis mirror with a parabolic figure will have a novel reflective coating of boron carbide that will be efficient in the extreme ultraviolet but let the solar visible and thermal radiation pass largely unaffected. In this way the heat load on the mirror is reduced. 79)
The scientific goal is to determine the plasma density, temperature, element/ion abundances, flow speeds and the structure of the solar atmosphere using spectroscopic observations of emission lines in the UV/EUV spectral region. Spectroscopic observations of emission lines in the UV/EUV region provide important plasma diagnostics of the solar atmosphere, providing the necessary tools for probing the wide range of solar plasma temperatures. These may range from tens of thousands to several million K. The analysis of emission lines, mainly from trace elements in the sun's atmosphere, provides information on plasma density, temperature, element/ion abundances, flow speeds and the structure and evolution of atmospheric phenomena.
The SPICE instrument consists of a single element off-axis parabolic telescope and a TVLS (Toroidal Variable Line Spaced) grating spectrograph with two IAPS (Intensified Active Pixel Sensor) detectors. SPICE also includes a DPU to control each of the mechanisms, perform data compression and provide the SpaceWire interface to the spacecraft. The off-axis parabola mirror forms an image of the Sun onto the entrance slit assembly containing three interchangeable slits of differing widths. The slit selects a portion of the solar image and passes it to a concave TVLS grating which re-images the spectrally dispersed radiation onto two array detectors. Beyond 0.35 AU, off-limb observations are made by inserting a quartz filter to reduce UV scattered light in the instrument and allow observations of the outer corona beyond >0.30 RS. The two spectral passbands cover the same spatial field of view simultaneously with no scanning of the detectors or grating. The detectors are solar blind, IAPS sensors, and require no visible light rejection filters. The stigmatic spectra produced are magnified, yet maintain high spectral resolution in one dimension and high spatial resolution in the other. The SPICE observing strategy is to produce 2D spectro-heliograms (spectral images) of selected line profiles and line intensities only. The wavelengths covered by SPICE are 702-792 Å (Band 1), 972-1050 Å (Band 2) and 485-525 Å (2nd order). The selected lines represent the full range of temperatures and heights in the solar atmosphere, from the chromosphere to the flaring corona. SPICE derives heritage from SOHO/CDS, SOHO/SUMER, as well as the RAISE and EUNIS sounding rocket programs.
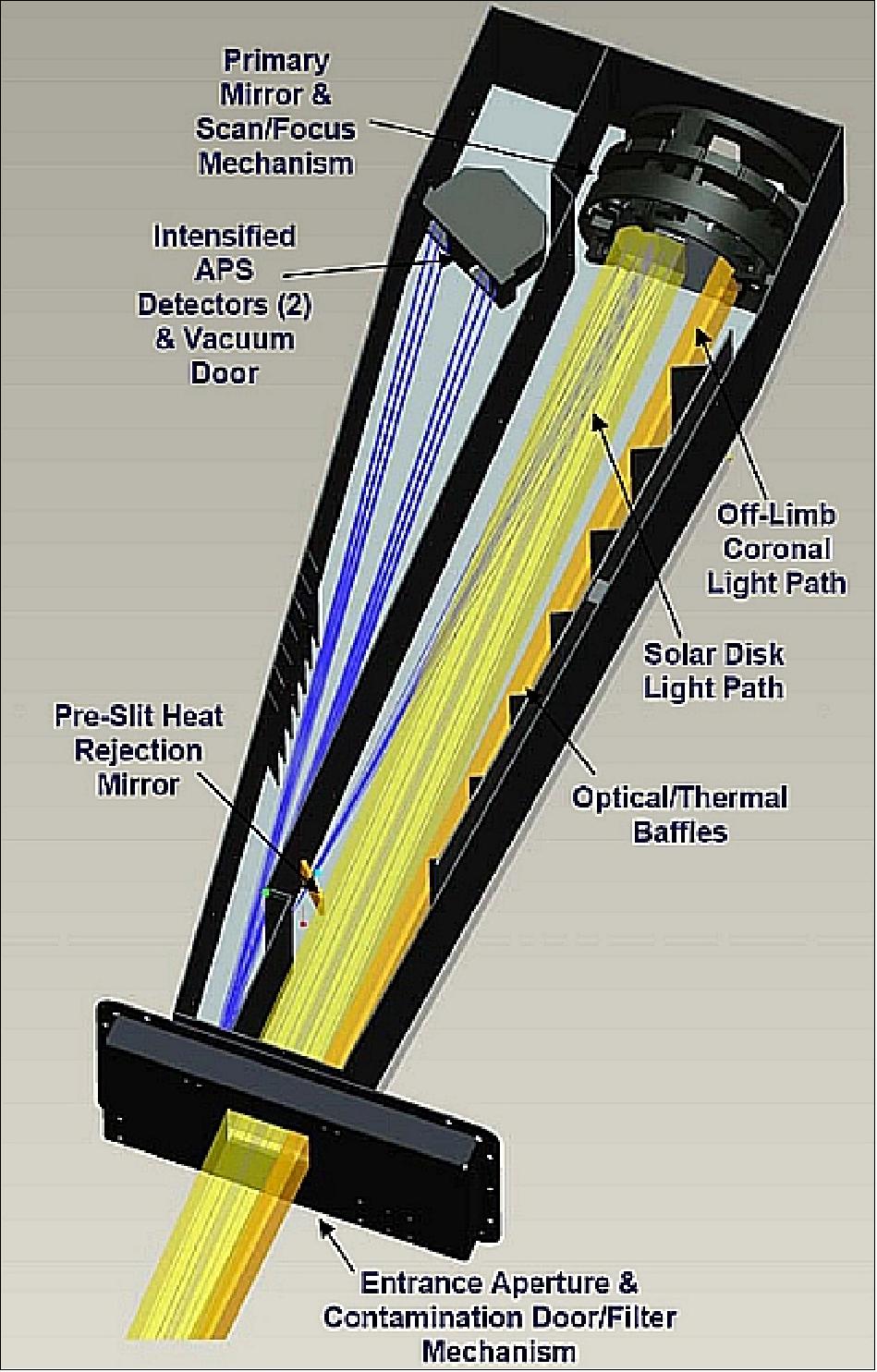
EUI (Extreme UV Imager)
The exceptional orbits of the Solar Orbiter mission permit to achieve breakthroughs in plasma astrophysics by imaging the magnetized solar atmosphere in the extreme-ultraviolet (EUV) emission.
The prime objectives of EUI are: 80) 81) 82)
• To provide EUV imagery with at least a factor 2 higher spatial resolution than currently available, to reveal the fine-scale structure of coronal features
• To provide full-disc EUV imagery of the sun to reveal the global structure and irradiance of inaccessible regions such as the ”far side” of the sun and the polar regions
• To study the connection between in-situ and remote-sensing observations.
The EUI instrument suite on board of Solar Orbiter is composed of two HRI (High Resolution Imagers), one at Lyman α and one dual-band in the extreme UV, and one dual-band FSI (Full Sun Imager) working alternatively at the two 174 Ä and 304 Ä EUV passbands. 83)
The EUI instrument is developed in a collaboration which includes CLS (Centre Spatial de Liège) and Royal Observatory of Belgium, Belgium, the IAS (Institut d’Astrophysique Spatiale) and Institut d’Optique, France, the UCL Mullard Space Science Laboratory (MSSL), London, UK, and MPS (Max Planck Institute for Solar System Research), Katlenburg-Lindau, Germany.
The Belgian institutions are funded by Belgian Federal Science Policy Office; the French institutions by CNES (Centre National d'Etudes Spatiales), the UK institution by PPARC (Physics and Astronomy Research Council); and the German institution by DLR (Deutsche Zentrum für Luft- und Raumfahrt e.V.).
The quiescent, large-scale corona can be imaged in the spectral bandpass centered at 174 Å and dominated by Fe IX/X emission lines formed at temperatures around 0.8–1.1 MK (million Kelvin). The lower transition region can be imaged in the 304 Å bandpass dominated by the He II emission line formed at a temperature around 0.08 MK (or lower). Simultaneity of images in two bandpasses is not required. Both bandpasses can thus be combined inside a single telescope. The telescope aperture of 0.5 cm allows achieving acceptable signal-to-noise ratios with exposure times between 1 - 10 s allowing a cadence of around 10 minutes in each bandpass (to allow the observations of solar eruptive events such as CMEs and flares).
Figure 105 shows the EUI optical unit with the main components of the three channels. The spectral selection is obtained by multilayer coating deposited on the mirrors and by a set of transmission filters rejecting the visible and infrared light. The detectors feature a 2 k x 2 k array for the HRI and a 3 k x 3 k array for the FSI channel. Back-thinned silicon CMOS-APS with 10 µm pixel pitch, sensitive in the EUV wavelength range are considered for the FSI and the HRIEUV channels. A front side illuminated CMOS-APS is considered for the HRILyα channel (Ref. 83).
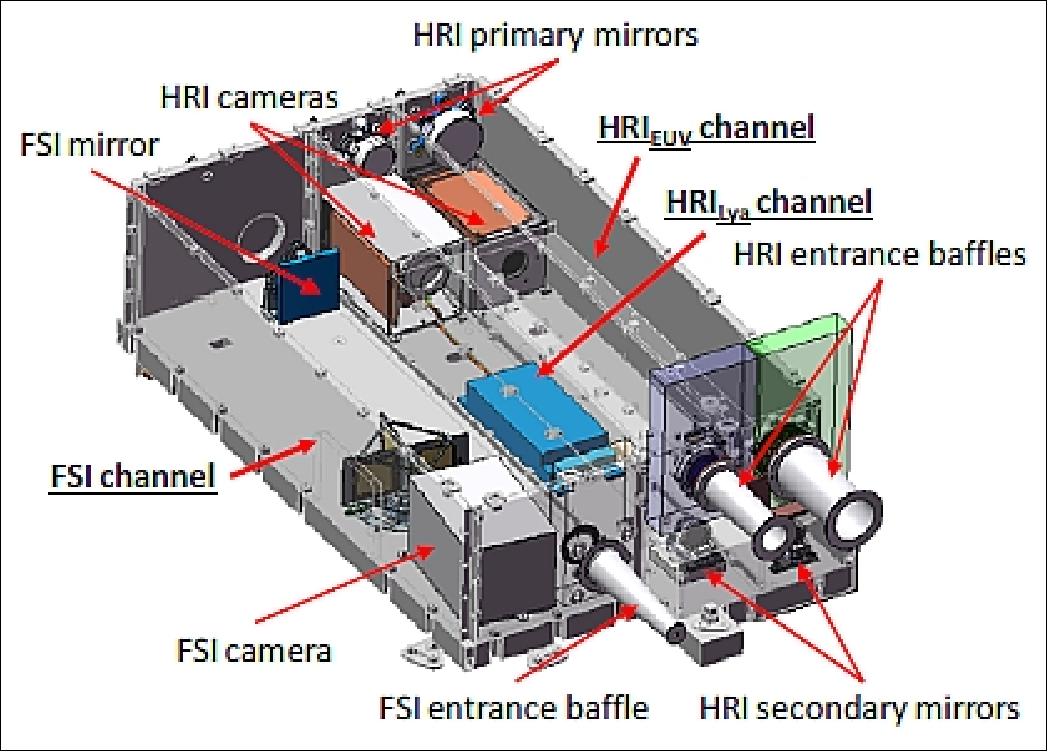
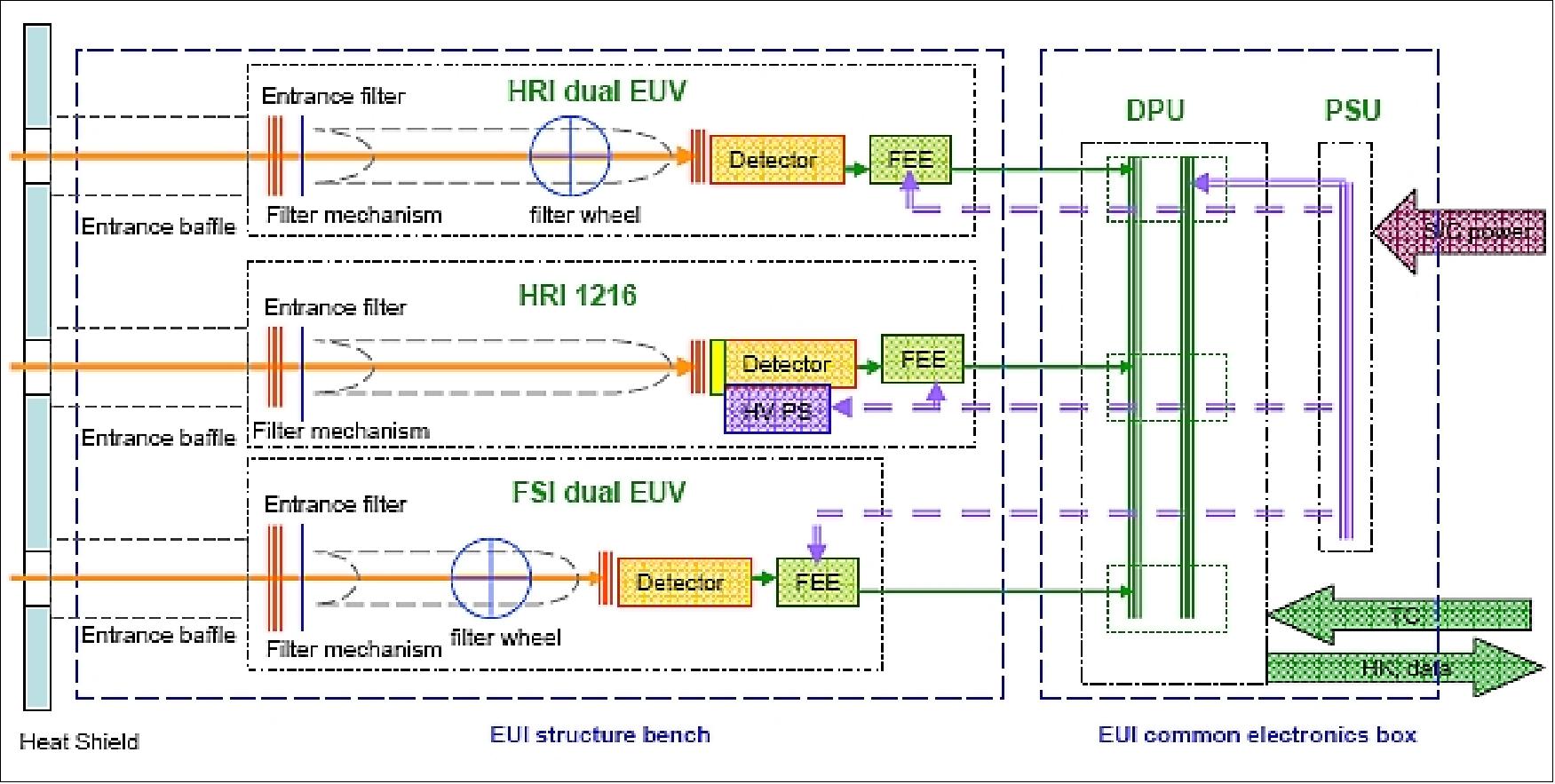
Parameter | Channel | Value |
Instrument dimensions |
| Optical bench: 900 mm x 600 mm x 230 mm |
Instrument mass (including margins) |
| 18.20 kg |
Nominal power consumption |
| 32 W |
Source data rate (telemetry) |
| 20.5 kbit/s |
Passband center | FSI dual EUV | 174 Å and 304 Å alternatively |
Passband center | HRI dual EUV | 174 Å and 335 Å alternatively |
Passband center | HRI Lyman-α | 1216 Å |
METIS (Multi-Element Telescope for Imaging and Spectroscopy) Coronagraph
The METIS instrument suite is conceived to perform both EUV (Extreme Ultraviolet) spectroscopy of the solar disk and off-limb, and near-sun coronagraphy and spectroscopy in FUV. METIS observations are crucial for answering some fundamental solar physics questions concerning the origins of the fast and slow wind, the sources of solar energetic particles, and the eruption and early evolution of coronal mass ejections.
The main scientific motivation of METIS is the attempt of addressing the three key scientific questions identified as the focus of the HELEX program: 84) 85) 86) 87) 88) 89)
1) The origin of heating/acceleration of the solar wind streams
2) The origin , acceleration and transport of the solar energetic particles
3) The transient ejection of coronal mass and its evolution in the inner heliosphere.
The METIS instrument suite consists of three different elements, sharing the same optical bench: electronics, power supply, and heat shield aperture. In particular METIS will provide for the first time:
- simultaneous imaging of the full corona in polarized visible light (590-650 nm) and narrow-band ultraviolet HI Lyman α (121.6 nm line)
- monochromatic imaging of the full corona in the extreme ultraviolet HeII Lyman α (30.4 nm line)
- spectroscopic observations of HeII Lyman α in corona.
The annular FOV (Field of View) ranges between 1.4 and 3.0 solar radii, when the perihelion is 0.28 AU, and the attained spatial resolution is 20 arcsec.
These measurements will allow a complete characterization of the three most important plasma components of the corona and the solar wind (electrons, protons, helium).
The three elements are identified as:
• COR (VIS and EUV Coronagraph)
• EUS (EUV Spectrometer)
• SOCS (Solar Orbiter Coronal Spectrometer).
The payload includes two core instrument packages, optimized to meet the solar and heliospheric science objectives.
METIS consists of a single optical head which uses a single aperture on the spacecraft sun facing thermal shield. The optical head is mounted on an optical bench (Figure 107), plus a main electronics and power supply box. The instrument front end consists of the visible-light and UV/EUV coronagraphic imager, which comprises the optics, detectors, proximity electronics and electrical interface. Internally to this coronagraph, in a suitable position, a dispersion grating intercepts a small portion of the solar corona (so that the obtained corona image is actually not complete) to perform UV and EUV spectroscopy. This additional channel does not need any mechanism, and shares one of the imaging coronagraph detectors.
Optical design: METIS is designed around an innovative concept for an externally occulted solar coronagraph, based on an IEO (Inverted External-Occulter). The IEO is a small circular aperture which replaces the classical annular aperture of the standard externally occulted solar coronagraph design. A boom connects the IEO to the M0, a small spherical mirror that rejects back the disk-light through the IEO. Many are the advantages of this novel design with respect to the classical one. Considering its application to this instrument, they can be summarized in the following points:
- smaller external occulter diameter
- thermal load on M0 greatly reduced
- on-axis telescope configuration
- more compact, cylindrical structure.
The smaller external occulter diameter implies a smaller aperture on the S/C thermal shield; the reduced thermal load produces a lower temperature inside the instrument and a better stability and control of the optical bench; the on-axis configuration gives a better optical performance, and has the advantage of a simpler mechanical structure; the compactness of the structure allows to optimize the available resources, and the cylindrical structure gives a symmetric configuration and an easier baffling, which is always an extremely critical point for solar coronagraphy. Beyond M0, an on-axis annular shape Gregorian telescope focuses the solar corona on the focal plane assembly. The Gregorian configuration of the primary and the secondary mirror, respectively M1 and M2, gives access to the primary focal plane for the placement of the IO (Internal Occulter). The IO blocks the light diffracted by the edge of the IEO and reflected by M1.
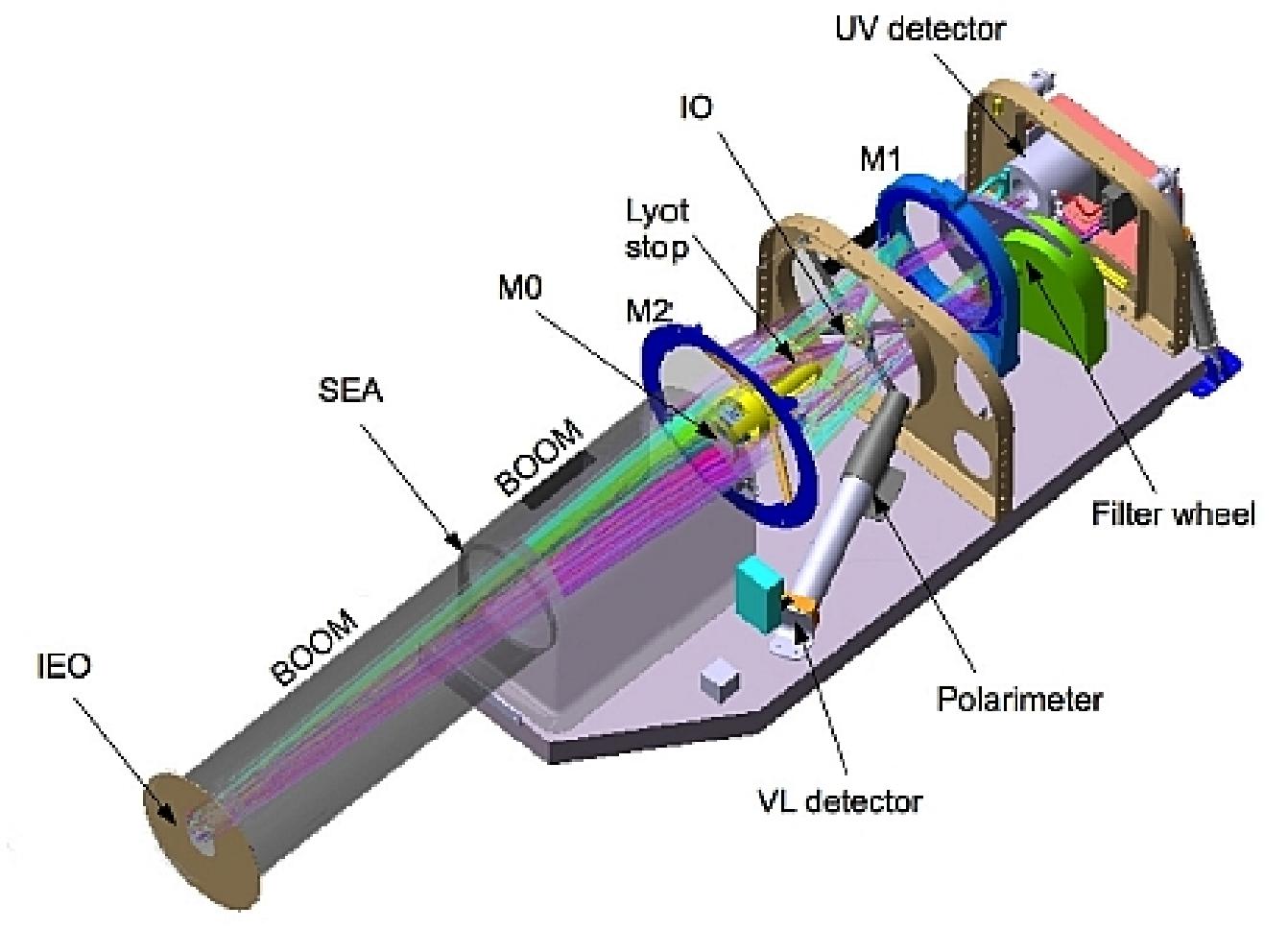
A conceptual scheme of the METIS coronal imager is shown in Figure 108. This is a novel design for an externally occulted all-reflective coronagraph, in which the external occulter has also the function of system entrance pupil. In such a way, the size of the external aperture is minimized and the thermal flux entering the instrument is greatly reduced, thus allowing to meet the stringent thermal requirements of SO. This novel optical configuration takes the name of ICOR (Inverted Coronagraph) because the diaphragms are inverted with respect to a standard coronagraph design.
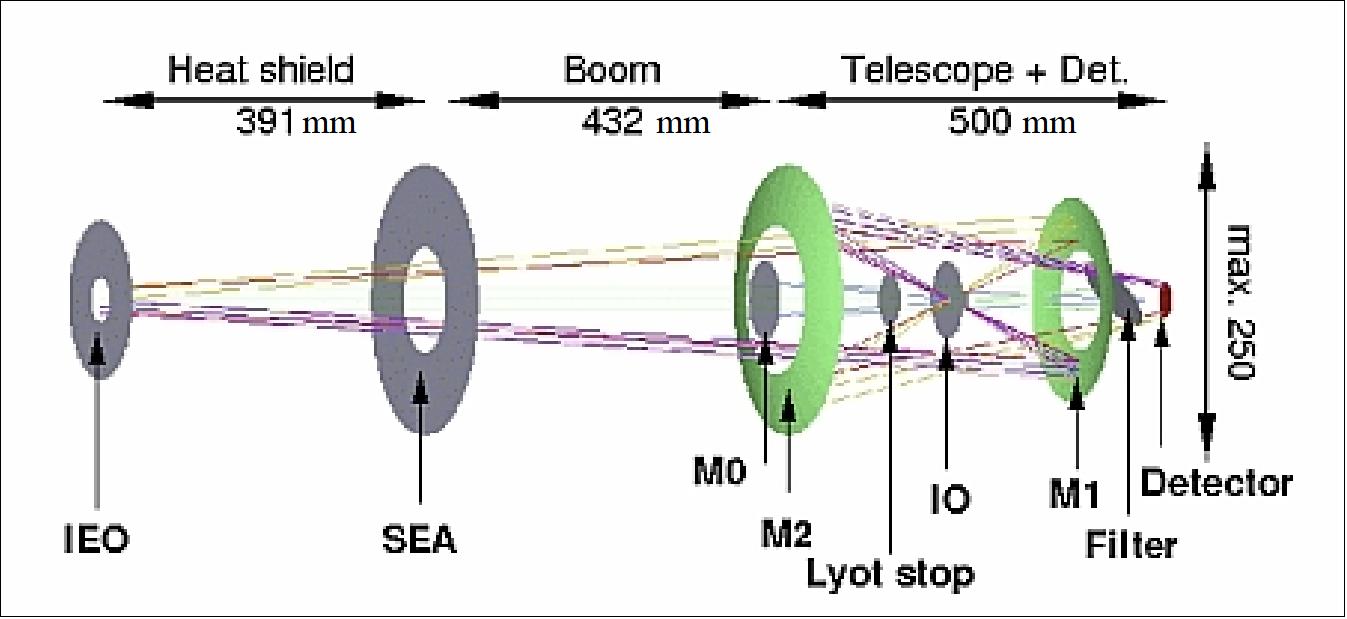
The UV/EUV and VL detectors are, respectively, an intensified active pixel sensor (IAPS) with scale factor 20 arcsec/pixel and image size: 30.7 mm (1024 x 1024) with 30 µm equivalent pixel size; and an APS with scale factor 10 arcsec/pixel and image size: 20.5 mm (2048 x 2048) with 10 µm pixel size.
Coronal imaging | |
Average instrument stray light (Bcor/Bsun) | VL < 10-9 |
Wavelength range | VL: 580-640 nm |
Effective focal length | VL: 200 mm; UV/EUV: 300 mm |
Spatial resolution | 10 arcsec (VL), 20 arcsec (UV/EUV) |
FOV (Field of View) | 1.5º - 2.9º annular, off-limb corona |
Temporal resolution | VL: 1-450 s |
Broadband linear polarization sensitivity | ≤ 10-2 |
SNR (Signal-to-Noise Ratio) | VL > 7 (7-590) |
Telemetry rate | 10.5 kbit/s |
Data volume (compression up to 10) | 27.2 Gbit/orbit |
Coronal spectroscopy | |
Wavelength range | UV: 121.6 ± 0.9 nm |
Spectral resolution | UV: 0.054 nm |
Spatial resolution | 34 arcsec |
FOV | Slit radial positions: 1.4º, 1.7º, 2.0º |
As shown in Figure 108, the first optical element is the IEO (Inverted External Occulter), which is in this design, a small circular aperture (40 mm Ø) in the Solar Orbiter thermal shield. Coronal light entering the IEO is then collected by a simple on-axis Gregorian telescope (aspherical mirrors M1 and M2), which makes the coronal image on the telescope focal plane where the detector is located. An IO (Inverted Occulter) is located close to the telescope prime focus with the function of blocking the light diffracted by the edges of the IEO. When METIS is pointing at the Sun's center, direct disk light impinges on a small (69 mm Ø) spherical mirror (M0) which backreflects it through the IEO. The portion between the IEO and M0 is called the “boom” and consists, optically, of three stops: IEO, on the front face of the S/C heat shield, and the SEA (Shield Entrance Aperture), on the back face of the S/C heat shield, and the annular aperture deliminated internally by M0 and externally by the hole inside M2.
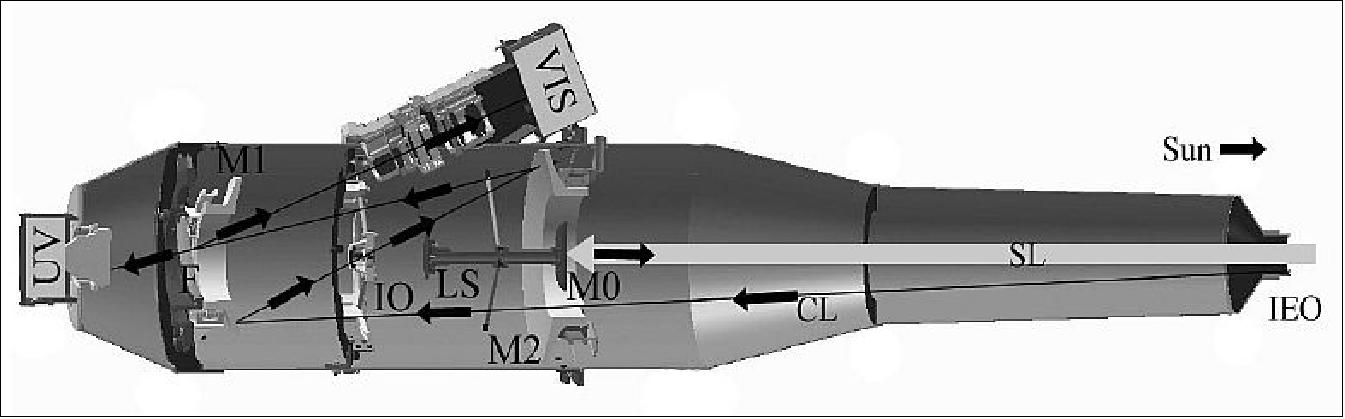
Some of the advantages resulting from this scheme with respect to the classical external occulter design are the following:
- Smaller diameter boom (~3 times) through the S/C thermal shield: this implies a smaller aperture on the S/C heat shield and an improved mechanical stability
- The thermal load on M0 is greatly reduced (by more than 90%) with respect to a standard externally occulted configuration: this means that there is a lower temperature inside the instrument, with a consequent easier control of the optical bench
- On-axis telescope configuration, which yields a better optical performance.
The telescope mirrors are coated with a suitable multilayer coating, optimized to enhance the reflectivity in the EUV He line, but still having good reflectivity at 121.6 nm and in the visible range. Hence, it is possible, by means of dedicated bandpass filters, to obtain the solar corona imaging at the three mentioned different wavelength bands.
A Lyot stop ,positioned slightly after the IO, blocks the light diffracted by M0 and reflected by M1. A filter wheel positioned just in front of the UV detector allows to select either visible and/or UV/EUV (HI 121.6 nm or HeII 30.4 nm line) images of the corona. When the thin aluminum filter is in the path, only HeII observations are performed. When the Al/MgF2 interference filter is in the path, the HI light is transmitted to the UV detector, while the broadband visible light (VL) is reflected towards the liquid crystal polarimeter, in order to perform measurements of the linear polarization of the visible solar corona.
To improve the scientific return of this instrument, a spectroscopic channel has also been included in the METIS optical path. Essentially, in the prime focus of the Cassegrain telescope, a three slit system is located in correspondence of an equatorial region of the solar corona; this slit system inhibits the possibility of doing imaging in this portion of the corona, so the actual coronal images of METIS will have a small sector missing. Light passing through the slits is collected by a diffraction grating located in a sector of the Cassegrain telescope secondary mirror. UV light is then dispersed and focused on the same UV/EUV detector used for imaging.
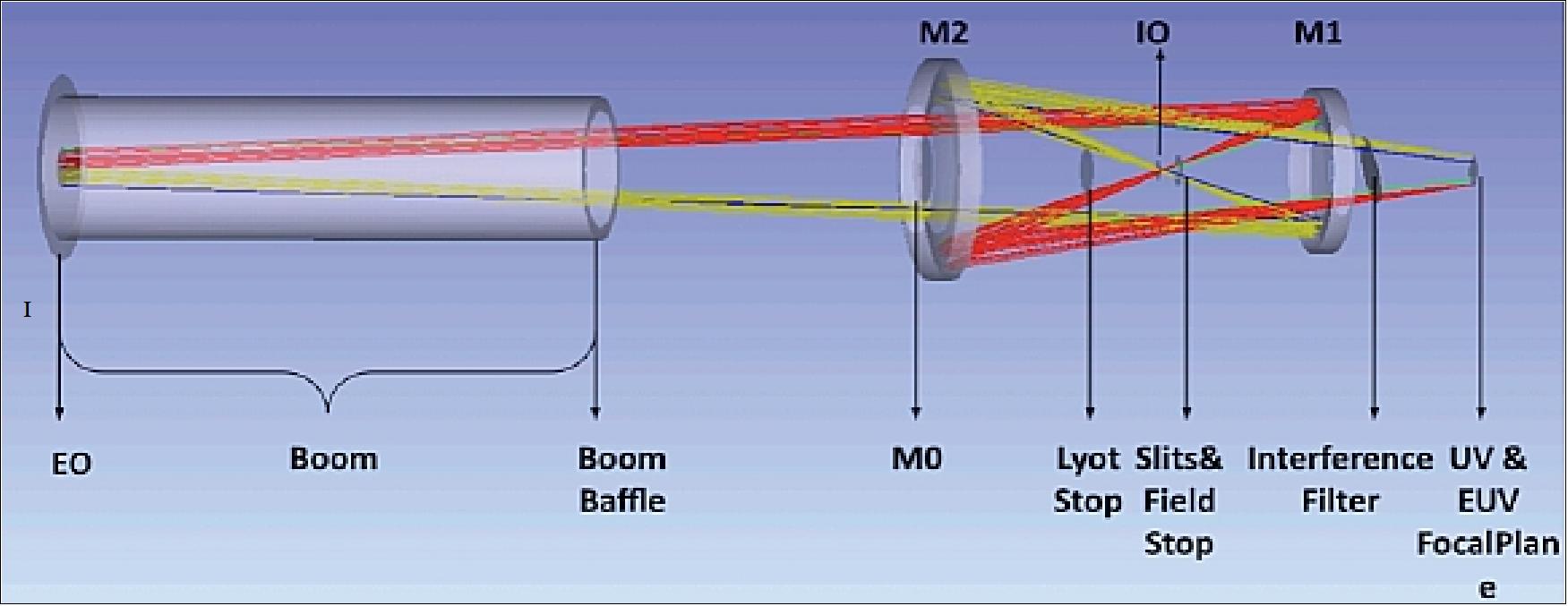
As an externally occulted coronagraph (Figure 110) the telescope has an occulting system between the Sun and the primary mirror, M1, that puts M1 in the shadow of the Sun disk. The rejection of solar disk radiation is the most critical issue in a coronagraph design: solar disk radiation that impinges on the coronagraph detectors, no matter how, is called stray light. Traditionally, the external occultation concepts consists of an occulting disk that prevents solar disk radiation from reaching the entrance pupil aperture. METIS operates with an inverted occulted system concept: light from the solar disk and from the corona enters a round external aperture that acts as the entrance pupil and is called IEO (Inverted External Occulter). Solar disk light is then blocked and rejected through IEO by a Sun disk rejecting mirror, M0. This configuration has the advantage, very important for this mission, of reducing the thermal load inside the instrument. In order to reduce the amount of stray light, the METIS Sun disk radiation is rejected by means of standard techniques used in externally occulted coronagraphs:
• a Sun disk rejection mirror, M0, that reflects back the disk radiation through the entrance pupil
• an internal occulter, IO, that is placed in the conjugate plane of the IEO relative to the primary mirror M1, that blocks light diffracted by the edge of IEO
• a Lyot stop, LS, placed in the conjugate plane of M0 relative to M1, that blocks secondary diffracted light from the edge of M0.
The stray light sources inside the coronagraph are due to two main contributors:
1) Sun disk light diffracted by the edges of the entrance aperture IEO Light diffracted by IEO is mainly taken care of by the internal occulter, IO, and by the Lyot stop, LS. Nevertheless, diffracted light impinging on the primary mirror, M1, is partially scattered by its surface and becomes the major contributor to the final stray light level.
2) Sun disk light entering the entrance aperture IEO Disk light entering IEO is taken care by the disk light rejection mirror, M0, that re-images the Sun disk back through IEO itself. Scattering from the M0 surface will contribute to the stray light level via diffusion off the telescope components: mounts and walls.
Additional contributions to the stray light will be produced by particulate contamination on those components illuminated directly by the Sun (IEO and M0) or illuminated by the light diffracted by IEO (M1, mounts and coronagraph walls).
An extensive theoretical analysis shows that the stray light levels in all three METIS wavelength bands meet the requirements. The analysis is based on an ideal instrument, i.e., it does not include manufacturing and alignment tolerances and also the cleanliness of the optical components, and includes approximations on the shapes on the occulters.
In order to characterize the coronagraph stray light, it is necessary to build a laboratory model of the stray light rejection subsystem and test it inside a special facility with the capability of reproduce as closely as possible the solar irradiance conditions on the coronagraph. Laboratory tests are important to better characterize the occulting system and find and improve its limits and defects (Ref. 87). Table 8 summarizes the sources of stray light and how METIS is dealing with them and Figure 111 shows the comparison between the stray light estimate at 0.28 AU of S/C heliospheric distance with the expected coronal signal.
Stray light source | METIS Stray light rejection |
Direct solar disk light | |
Sun disk light entering IEO (Inverted External Occulter) | Reflected back through IEO by M0 |
Sun disk light scattered by M0 | Reduced by black coating of the boom |
Sun disk light scattered by S/C components above IEO | Reduced by black coating on IEO |
Sun disk radiation diffracted by IEO | |
Sun disk diffracted by IEO and reflected by M1 | Blocked by IO |
IEO diffraction, diffracted by M0 | Blocked by Lyot stop |
Sun disk diffracted by IEO and scattered by M1 | Minimized with very highly polished M1 |
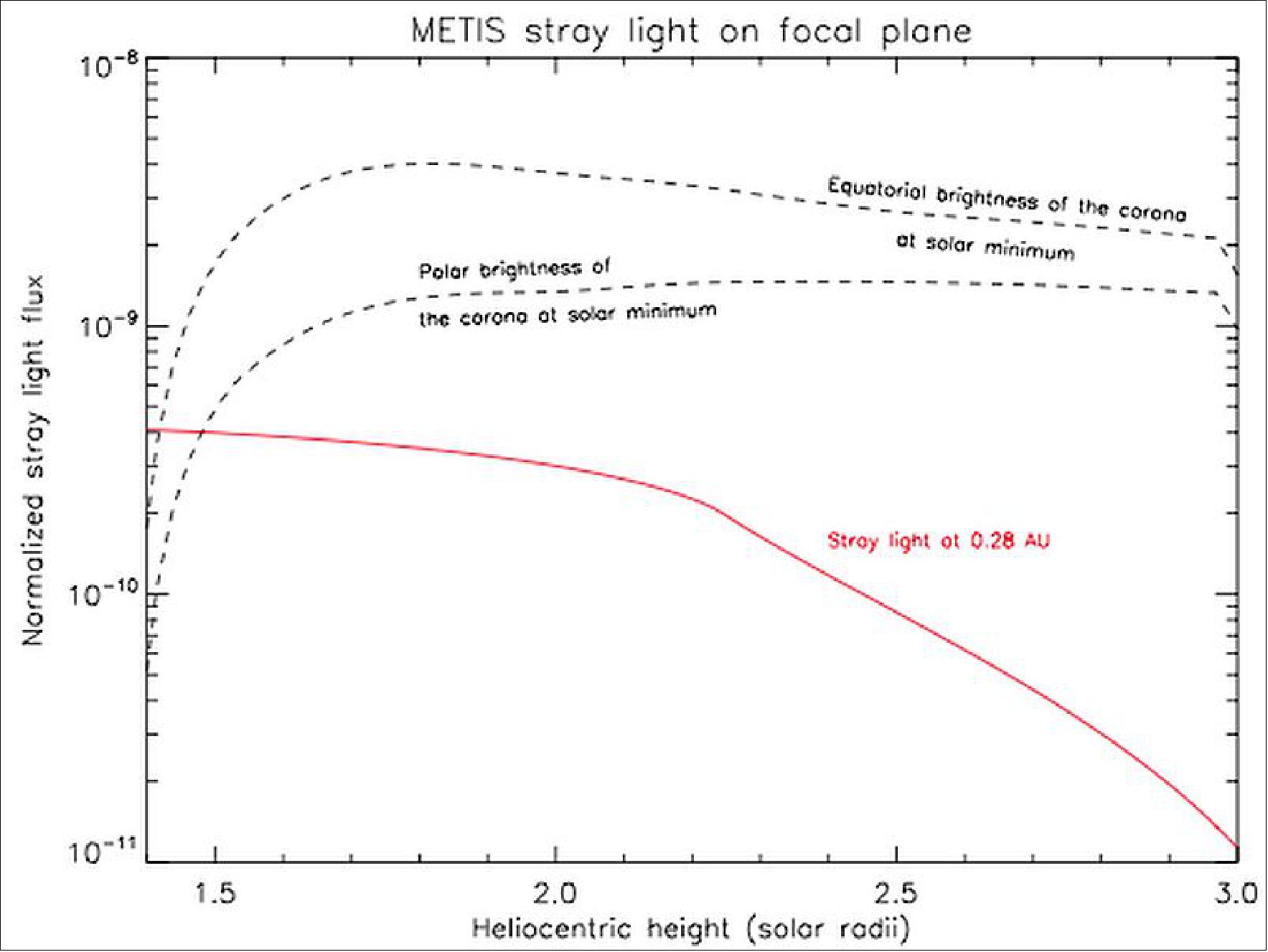
METIS In-flight Off-pointing
The Solar Orbiter mission profile foresees three 10 day length observing periods per orbit for the remote sensing instruments which are demanding in terms of resources, included telemetry. Each period is called RSW (Remote Sensing Window) and includes the spacecraft pass through perihelion and maximum and minimum heliolatitude.
Outside the RSWs, the RS instruments do not operate and the METIS door will be closed. During the RSWs, the Solar Orbiter will be sun-center pointed with the exception of planned off-pointing windows to allow high resolution instruments to point targets on the disk up to the limb, and of S/C maneuvers such as wheel off-loading, high gain antenna maneuvers, breaks in fine guidance polynomials.
METIS, as a coronagraph, is designed to operate sun-centered. When the spacecraft is off-pointed, METIS can operate with degraded performance up to a limit of off-pointing which is a function of the instrument parameters and of the heliocentric distance of the Solar Orbiter.
Two limit angles, αmax and βmax, are defined, respectively, as the maximum angle of off-pointing at which, with degraded performance, it is possible to acquire scientific data, and as the maximum angle at which safety procedure are needed to protect the instrument.
As shown in Figure 112, αmax is the angle at which the solar disk radiation starts to illuminate the lateral surface of the IEO cone, while βmax is the angle at which M0 does not block the full disk light. Figure 112 provides also the numerical values of αmax and βmax, while Figure 113 shows the dependence of these angles from the S/C heliocentric distance. Both angles take into account the APE (Absolute Pointing Error) of both the S/C and METIS. The Figure 113 displays also the size of the solar disk as a function of distance, showing that about 0.55 AU, the maximum allowed off-pointing exceeds the size of the Sun.
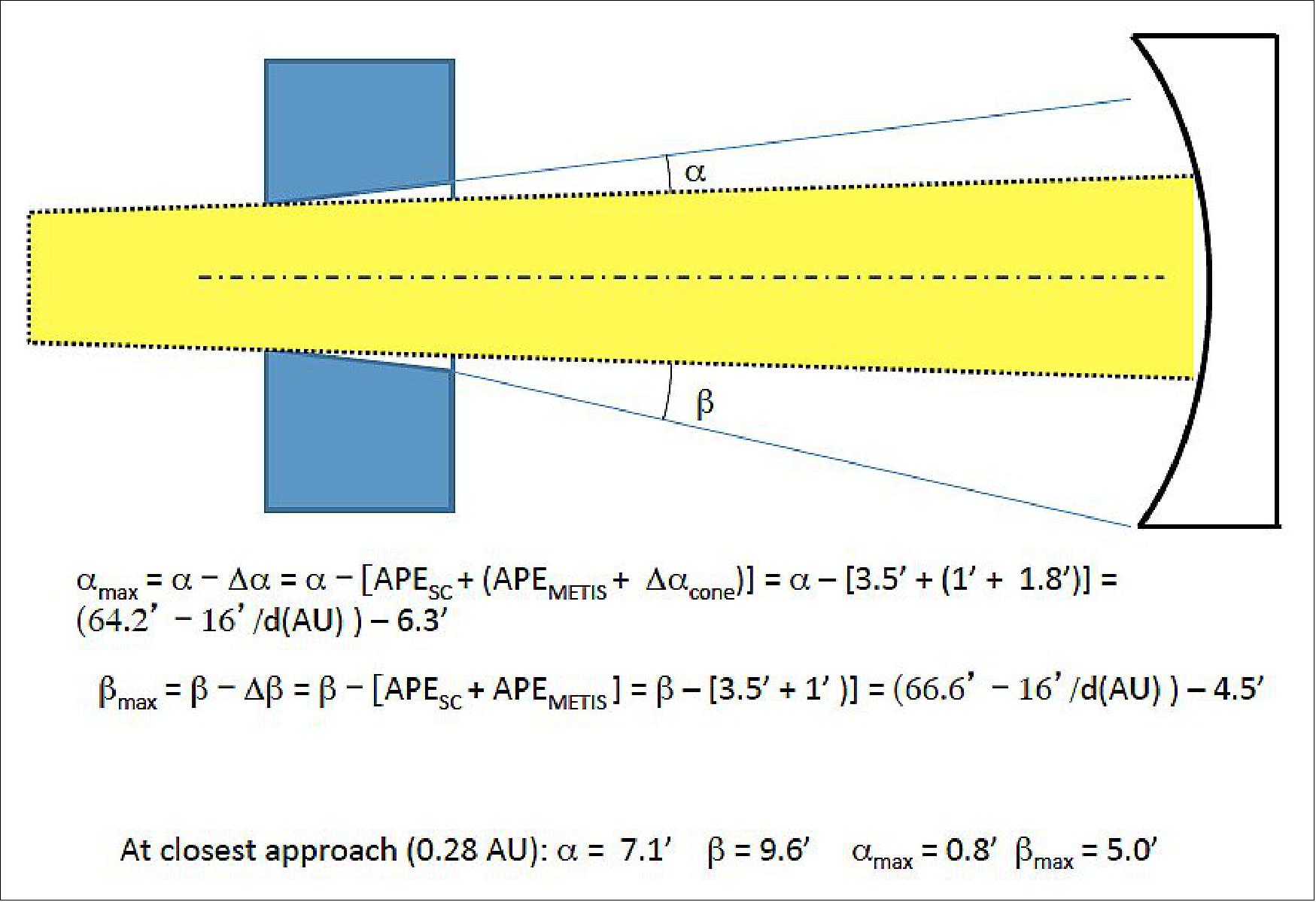
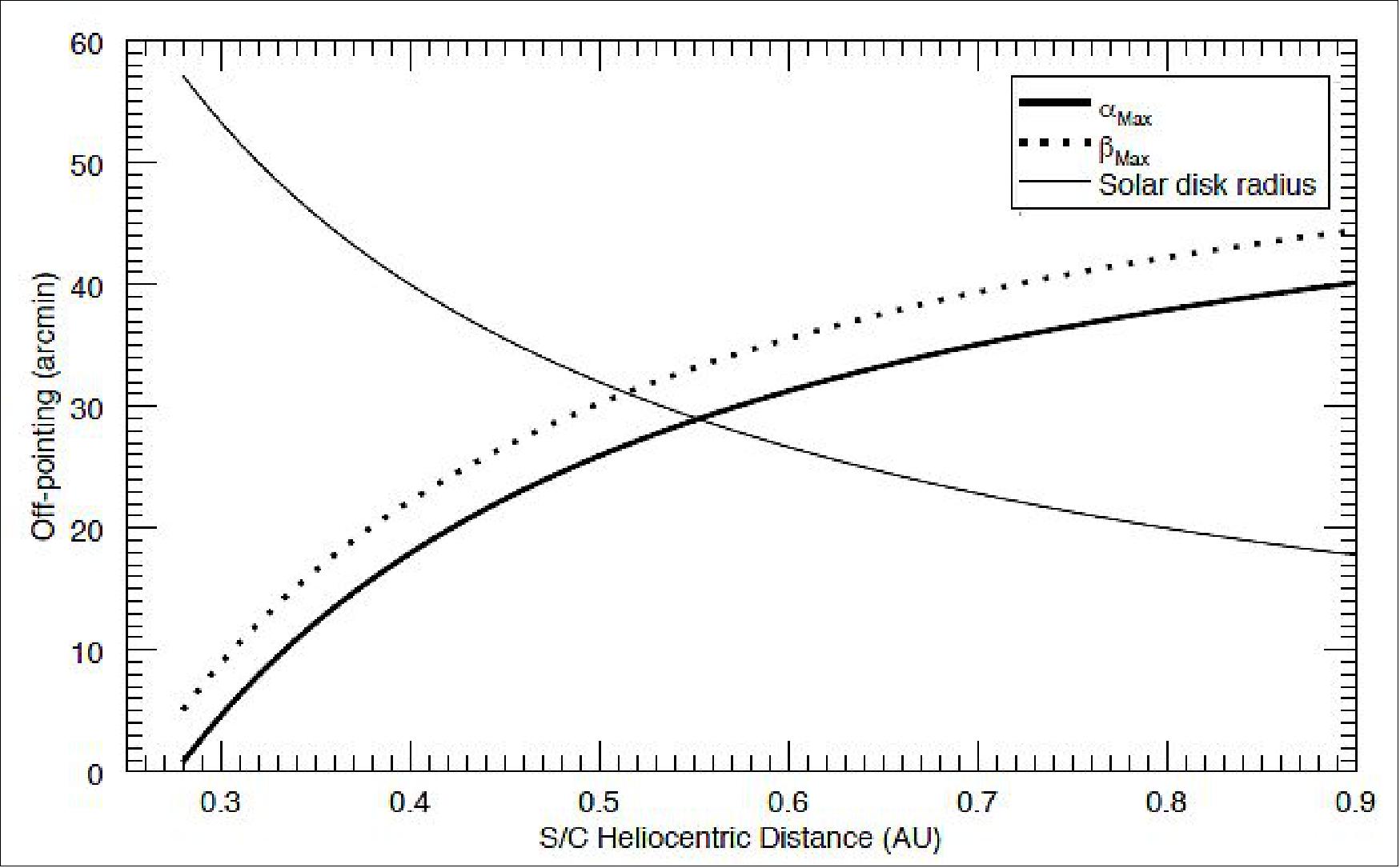
METIS Operating as a COR (Coronagraph)
Co-rotation during the helio-synchronous phases of the orbit will freeze coronal structures in the plane of the sky for many days. This allows the study of the evolution of the magnetic configuration of streamers to test the hypothesis of magnetic reconnection as one of the main processes leading to the formation of the slow solar wind. The out-of-ecliptic vantage point will also allow a unique view of the plasma distribution and solar wind expansion in the coronal low-latitude/equatorial belt. Therefore, it will be possible to measure the longitudinal extent of coronal streamers and coronal mass ejections. These parameters, that at present are unknown, are essential to determining the magnetic flux carried by plasmoids and coronal mass ejections in the heliosphere.
The prime objectives of COR are:
• To investigate the evolution of the magnetic configuration of streamers in order to test the hypothesis of magnetic reconnection as one of the main processes leading to the formation of the slow solar wind during the quasi helio-synchronous phases of the orbit
• To measure the longitudinal extent of coronal streamers and coronal mass ejections from an out-of-ecliptic advantage point. These data are essential to determine the magnetic flux carried by plasmoids and coronal mass ejections in the heliosphere
• To investigate the large-scale structure of the F-corona (the dust) and the cometary sources of the dust near the Sun. This will provide important information for the in-situ instruments in the payload that measure plasma and dust.
Instrument concept: COR is an externally occulted telescope designed for broad-band polarization imaging of the visible K-corona and for narrow-band imaging of the UV corona in the H I Lyman-α, 121.6 nm, line in an annular field of view between 1.2 and 3.5 solar radii, when the Solar Orbiter perihelion is 0.22 AU.
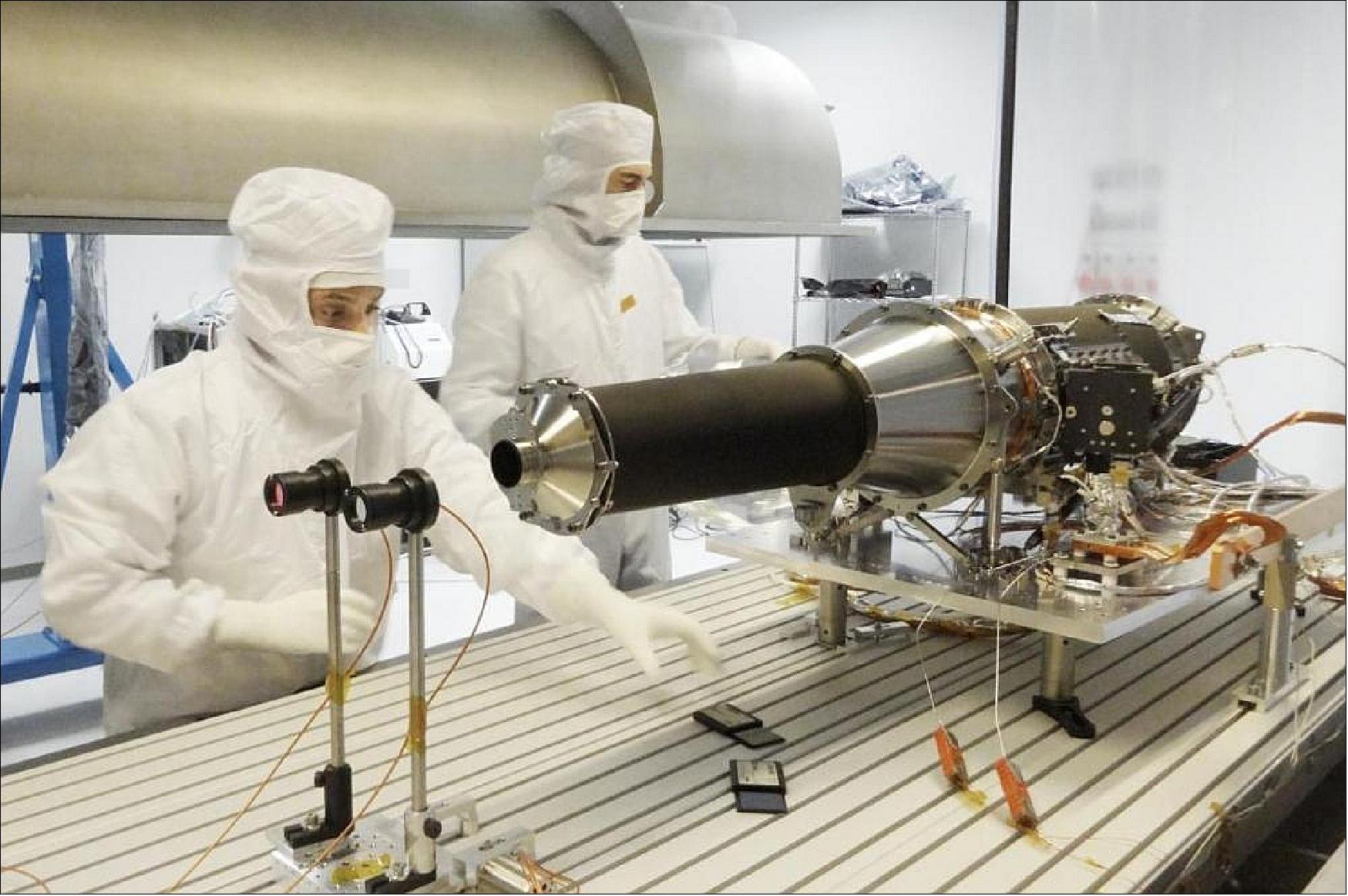
STIX (Spectrometer / Telescope for imaging X-rays)
STIX is a state-of-the art X-ray imaging telescope for solar observations designed and built at FHNW (Fachhochschule Nordwestschweiz, Windisch - University of Applied Sciences and Arts Northwestern Switzerland). STIX provides imaging spectroscopy of solar thermal and non-thermal X-ray emission, measurements at the highest ever spatial resolution and sensitivity. The PI (Principal Investigator) of STIX is Arnold Benz of ETH Zürich. 91) 92) 93)

Bursts of hard X-rays (> 20 keV) are the most common signature of the impulsive phase of a solar flare. In fact, the X-ray continuum is the most direct signature of energetic electrons at the Sun. The X-rays are bremsstrahlung, produced by accelerated electrons colliding with the ambient solar atmosphere. STIX is an X-ray imaging spectrometer, operating from 3 to 150 keV, which determines the location of X-ray emission from the sun as a function of time and energy to a spatial precision of 1 arcsec over a 38 arcmin imaging FOV and 2 arcmin over a wider 5º FOV.
The prime scientific objectives of STIX are: To establish the timing, location and spectra of energetic electrons near the sun. This will enable these electrons to be related to subsequent observations by the in-situ solar energetic particle and radio instruments. In this way, STIX serves as a high-energy link between imaging and in-situ observations. In conjunction with in situ instruments onboard both SolO and SPP, STIX will analyze the magnetic link between the heliospheric electron populations and their host regions of injection, close to the Sun. Additional STIX science objectives are: 94)
1) To determine the size and morphology of hot (>10 MK, formed by solar flaring activity) thermal plasma and non-thermal hard X-ray sources on the Sun with a spatial resolution of 400 km at closest perihelion, about 5 times better than previously achieved
2) To compare with co-temporal observations of other space-based instruments in order to measure the directivity of solar X-ray emission
3) To compare observations of partially occulted, ”behind-the-limb” solar flares with those of other possible spaceborne instruments in order to isolate weak coronal components of hard X-ray emission from the bright footpoint sources and hence determine the relationship between energetic electrons that lose their energy in the corona and those that impact the solar chromosphere.
Instrument concept: STIX imaging uses the same indirect imaging technique as employed on Yohkoh / HXT. Imaging information is encoded in the relative count rates in separate detector elements located behind pairs of grids, each of which absorbs a distinct directionally-sensitive fraction of the incident flux. The telescope employs a set of 64 sub-collimators, each of which consists of a pair of widely separated, X-ray opaque grids with an X-ray detector element located behind the rear grid. Front and rear grid pairs have identical pitch and orientation, whose choice determines the spatial frequency to be measured.
As was demonstrated by Yohkoh/HXT, the relative count rates of a pair of sub-collimators, one of whose grids is displaced by one quarter of its pitch, can be used to accurately measure both the real and imaginary parts of one Fourier component of the angular distribution of the source. With 64 sub-collimators, the imaging system then measures 32 different Fourier components. This data can then be used to reconstruct the source image, using well-established techniques employed by radio astronomy, Yohkoh/HXT and RHESSI.
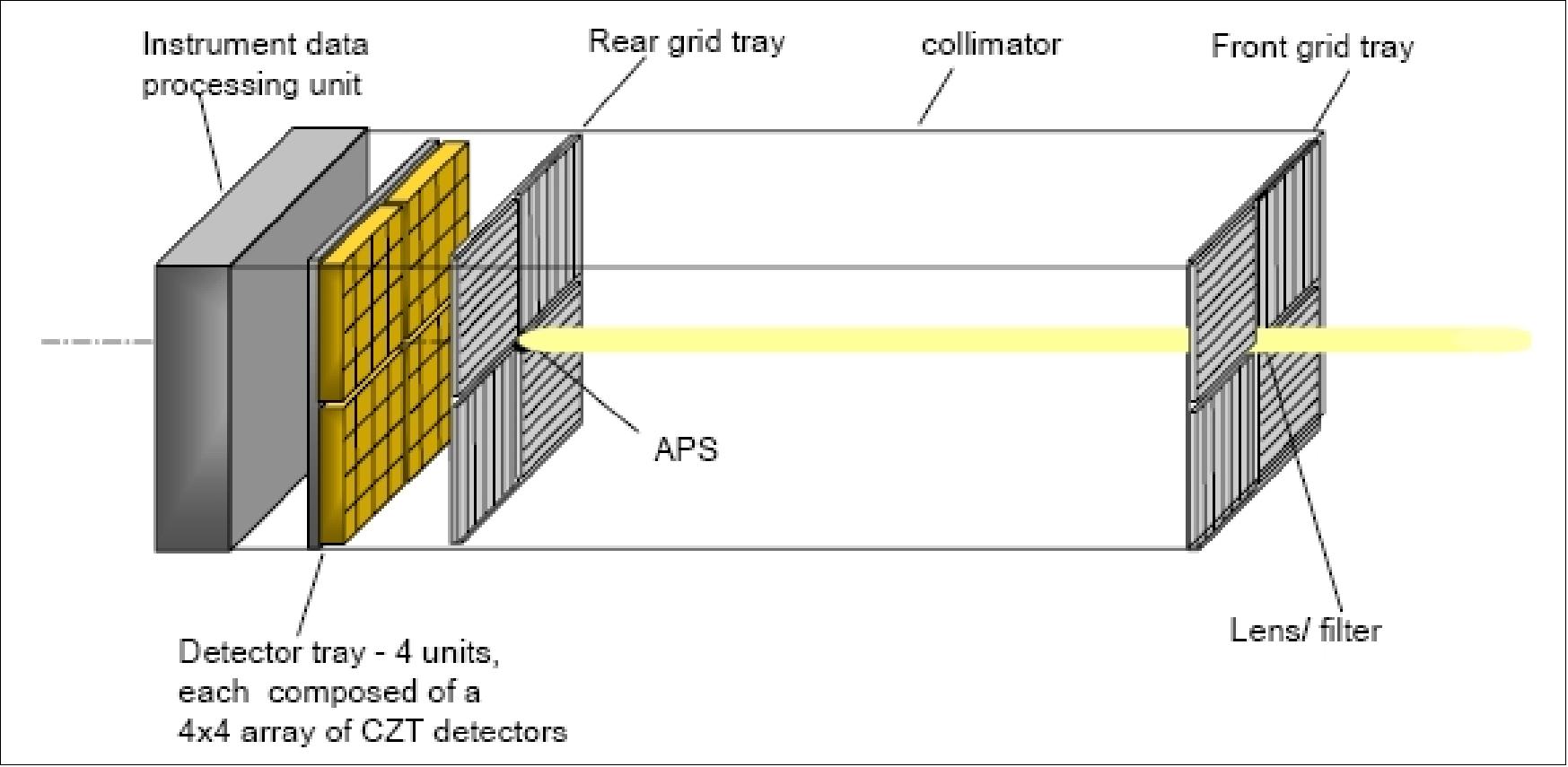
The two main parts (i.e., the imager and the electronics box) of STIX are mounted independently on the spacecraft. The imager consists of 32 pairs of X-ray opaque grids mounted in front of 32 solid-state CZT (Cadmium Zinc Telluride) X-ray detectors which are located on the electronics box and make up the spectrometer. 95)
STIX will cover the energy range between 4 keV and 150 keV with an energy dependent resolution of 1 keV to 15 keV. Its finest angular resolution and temporal resolution are 7 arcsec and 0.1 seconds, respectively. The transmission of each grid pair is very sensitive to the direction of incidence of the X-ray flux. The relative count rates of the detectors behind the different subcollimators (different pitches & orientations) encode the spatial information.
• 32 pairs of subcollimators measures 32 spatial Fourier components • Grid pitch and orientation → spatial frequency that is measured. • Images reconstructed by Fourier inversion, MEM, CLEAN, etc. via ground-based software. Heritage from: Yohkoh/HXT, RHESSI. Location to ~1 arcsec level. • STIX can also provide flare trigger and determine approx. flare locations (~1–3 arcmin) on-board for possible inclusion in real-time beacon-mode telemetry. • Field of view for imaging: 1.5º (full Sun @ 0.33 AU), locating: 2.5º (full Sun @ 0.23 AU) |
• Cadmium Zinc Telluride (CZT) - X-rays create electron-hole pairs in crystal - Bias voltage → pulse proportional to energy loss - Operate at room temperature • Four 4 x 4 arrays of 1 cm x 1 cm x 5 mm CZT detectors, one behind each subcollimator • Approx. energy range ~4 to 150 keV • Energy resolution ~1–15 keV • 32 logarithmically-spaced energy channels |
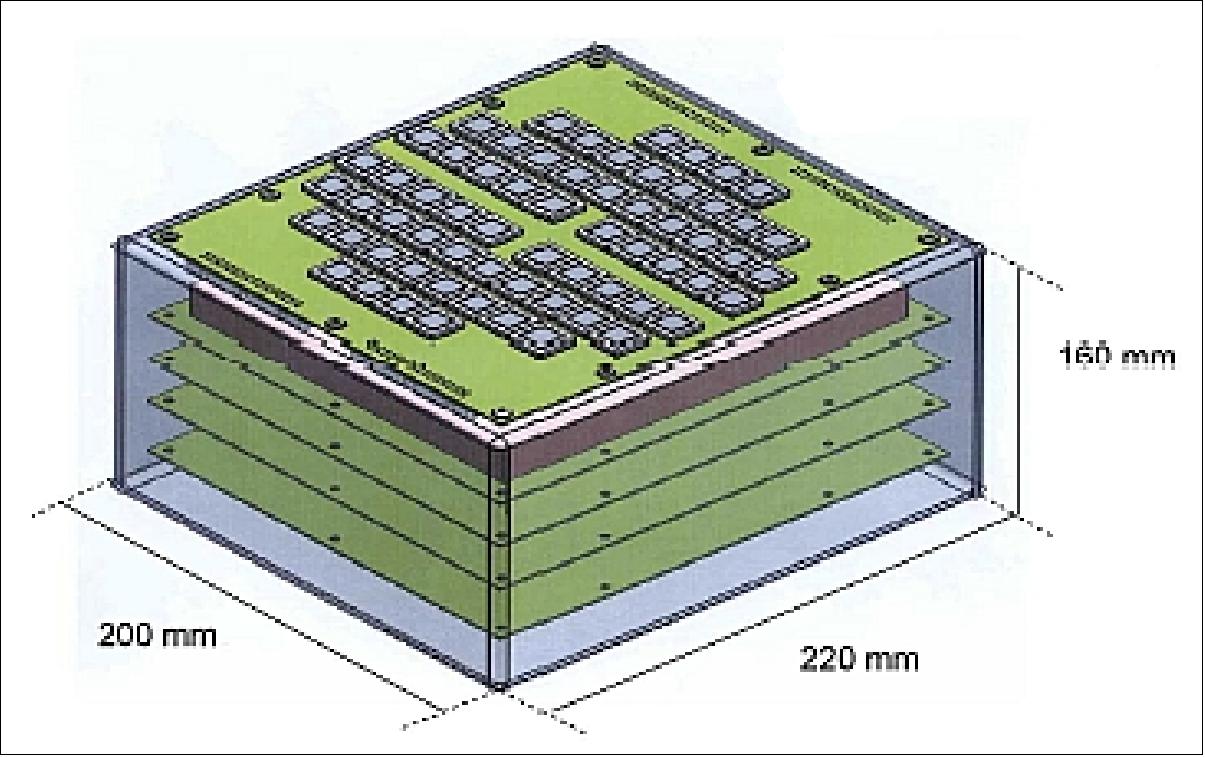
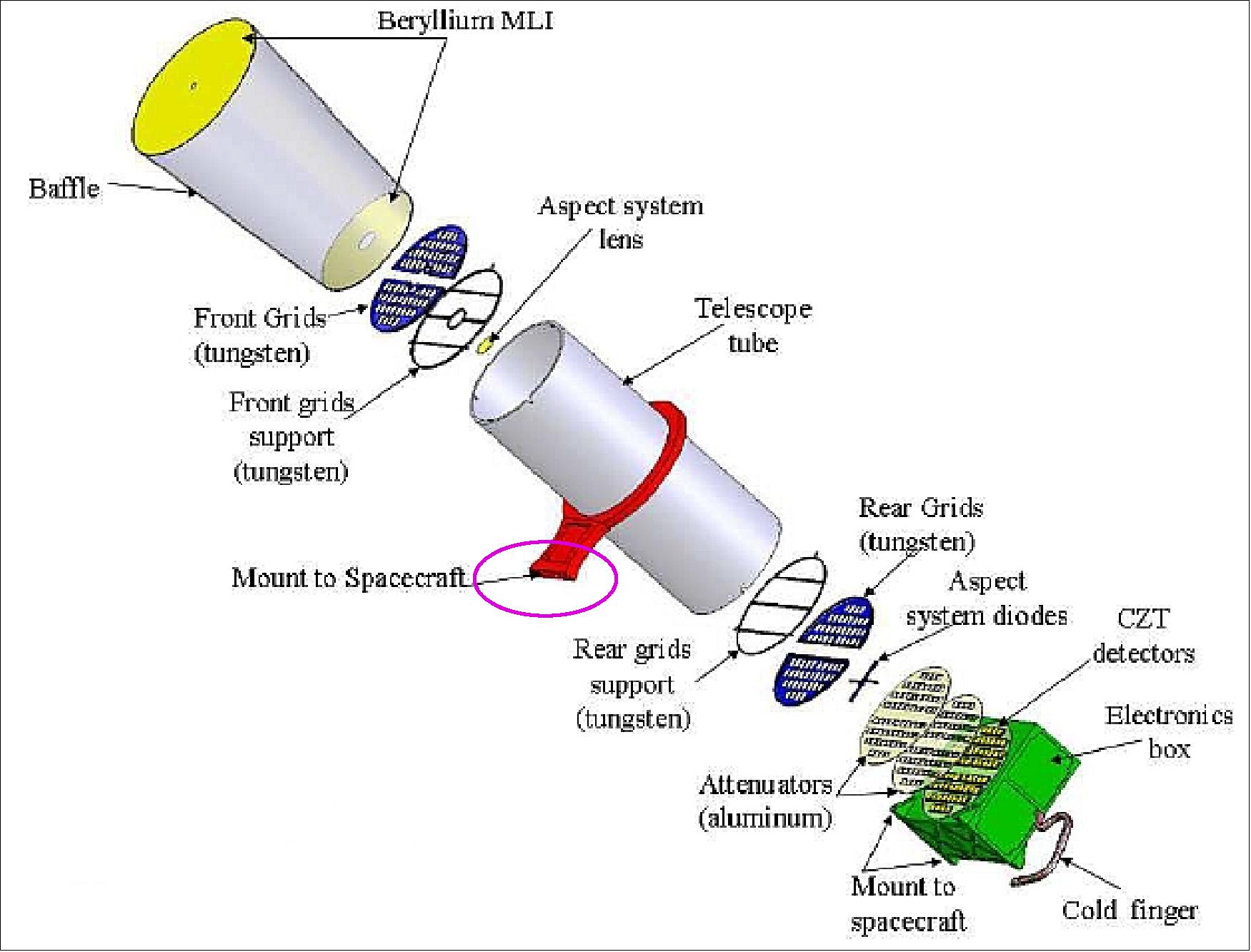
Legend to Figure 118: The attenuators are encircled. They are movable and are automatically inserted between the rear grids and the detectors during large flares to prevent excessive count rates at low X-ray energies. The attenuators will be developed by the Greek team (Ref. 94).
STIX attenuators: The attenuators, or shutters, which will be developed by the Greek team, are a critical component of the STIX instrument (Figure 118). Attenuators are dictated by the substantial dynamical range of incident X-ray fluxes from solar flares: the largest X-ray flares can provide as many as 105 more count rates in X-ray photons compared to those of the smallest micro flare that STIX can detect. For steep flare spectra and for fluxes between 3 - 150 keV, count rates can be higher by factors of 107 to 109. - An additional factor of 20 must be accommodated because of SolO’s varying heliocentric distance from successive aphelia to perihelia. The attenuators must be movable, enabled at high count rates and disabled at lower ones to protect the STIX detectors from excessive low-energy signal and at the same time allowing them to retain full sensitivity to small flare events. This will make STIX responsive to the entire dynamical range of solar X-ray flux (Ref. 94).
STIX will include two movable attenuators, mounted on the sunward side of the spectrometer module. Each of them will consist of a circular aluminum sheet with different widths (thick and thin). Both sheets are known to preferentially absorb a known fraction of the low-energy X-ray flux - the flux mostly responsible for the excessive signal - incurring a negligible effect at higher X-ray energies.
The movable attenuators will be complemented by a fixed insertion mechanism that will dictate their motions. The insertion mechanism will be triggered into action by an electronic signal depending on the incident count rate of X-ray photons. The signal will rely on internal logic and will generate an electric pulse without spacecraft-generated commands for the insertion or removal of the shutters. The shutters will be ordered to move one at a time by a software-generated command when the incident X-ray flux exceeds a preset threshold. This software, allowing the STIX processor to command one or both shutters to shift from one fixed position to another to attenuate the low-energy X-rays will be contributed by a Czech team participating to the STIX consortium.
STIX science (Ref. 91):
1) Field line tracking: STIX X-ray observations, in combination with other SolO instruments, in particular radio and >keV electrons measurements, will be used to magnetically link the heliospheric region where SolO is making in-situ observations back to regions at the Sun. This directly addresses a prime SolO science question: “What are the origins of the solar wind streams and the heliospheric magnetic field?”
2) X-ray imaging spectroscopy: STIX measurements of the characteristics of the energetic electrons at the Sun can be directly compared to EPD’s (Energetic Particle Detector) measurements of the electrons in-situ at SolO, to address another prime science question: “What are the sources, acceleration mechanisms, and transport processes of solar energetic particles?”
• Stereo observations between STIX and other X-ray instruments
- Direct measurement of source height (by comparing positions)
- Directivity of electrons (by comparing intensities)
- Isolate and characterize coronal contributions and relate to footpoint emission (difficult to do with normal imaging)
3) X-rays from CMEs (Coronal Mass Ejections):
• X-ray observation of disk-occulted events: STIX’s high sensitivity measurements of X-rays from the very high coronal sources associated with fast CMEs can help to address another prime question: “How do coronal mass ejections evolve in the inner heliosphere?”
• HXR (Hard X-Ray) emission from electrons in magnetic structures related to coronal mass ejections.
4) High sensitivity studies:
• Effective area ~ 0.25 RHESSI → ~ 5 times RHESSI at 0.23 AU
• Lower background than RHESSI → 2.5 times smaller events can be seen
• Up to 15 times more sensitive to faint emission
- HXR micro/nanoflare observations (coronal heating)
- HXR emissions from quiet Sun
- HXR emissions related to CMEs (in occulted events)
- HXR emission from escaping electron beams
- Axions
Instrument mass, power | 7 kg, 4 W |
Energy rage, energy resolution | 4-150 keV, 1 keV (FWHM @5 keV) |
Effective area | 6 cm 2 |
Angular resolution | 7 arcsec |
Pointing accuracy | 4 arcsec |
FOV (Field of View) | 2º |
Time resolution | 0.1s (statistics limited) |
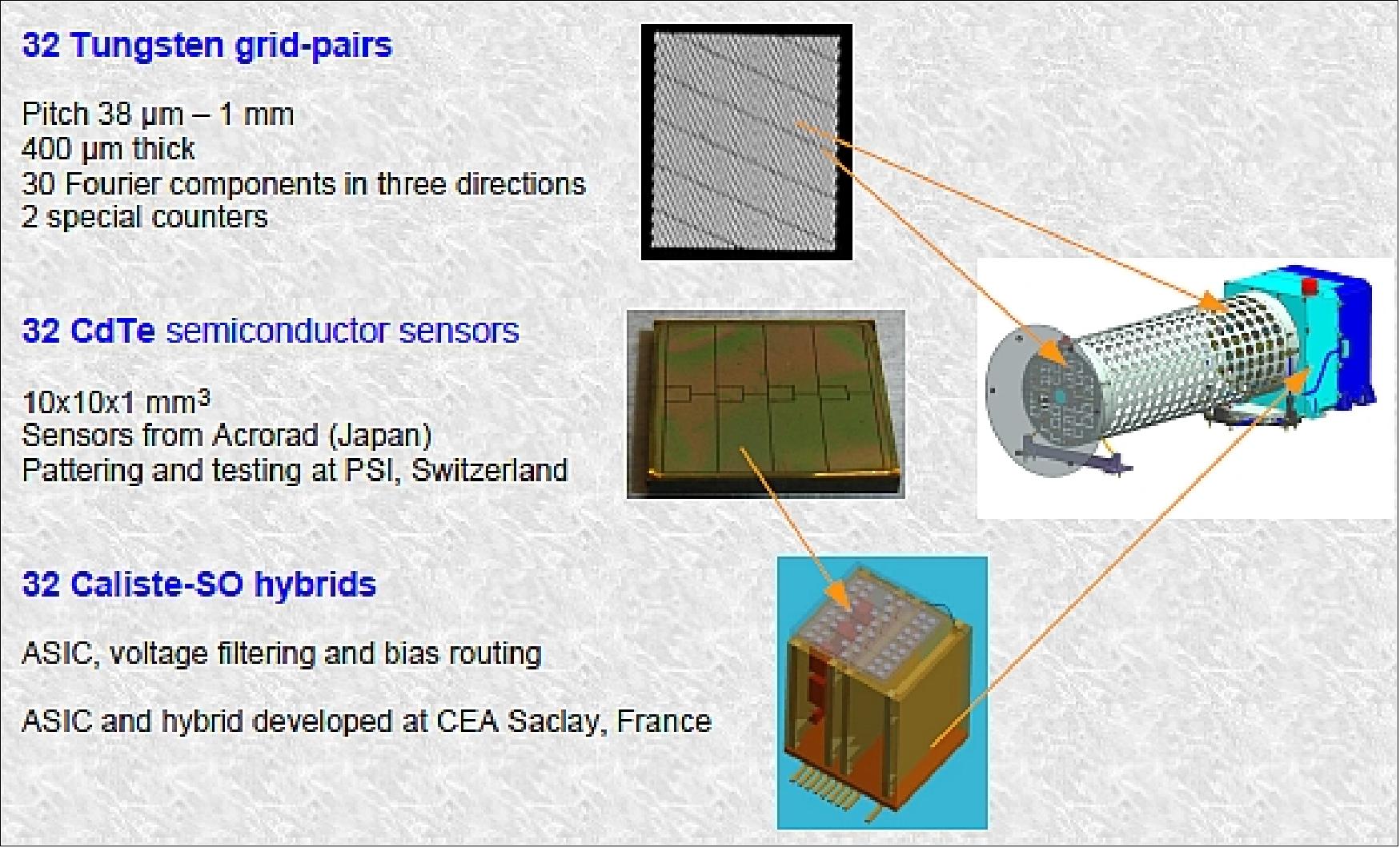
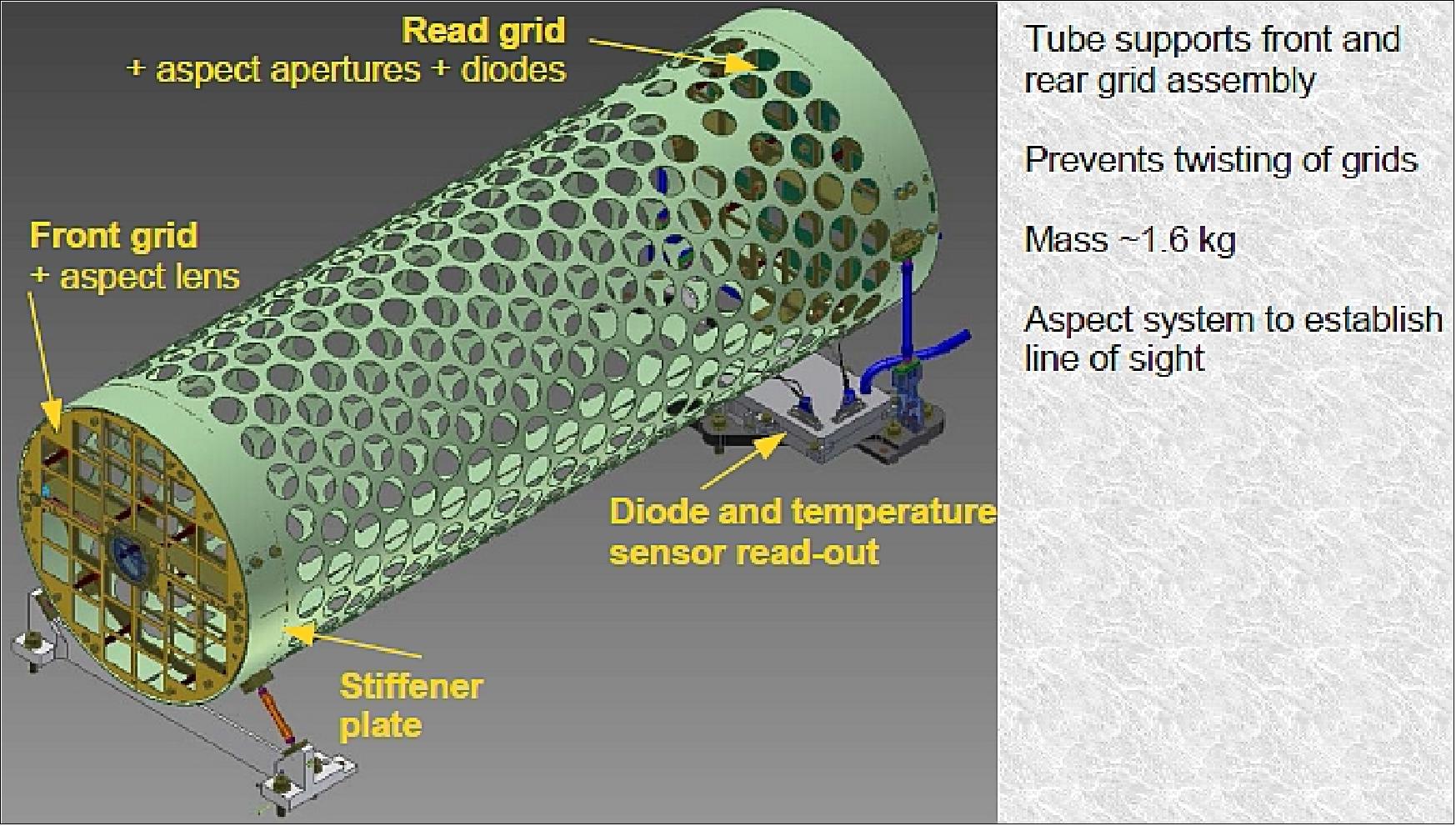
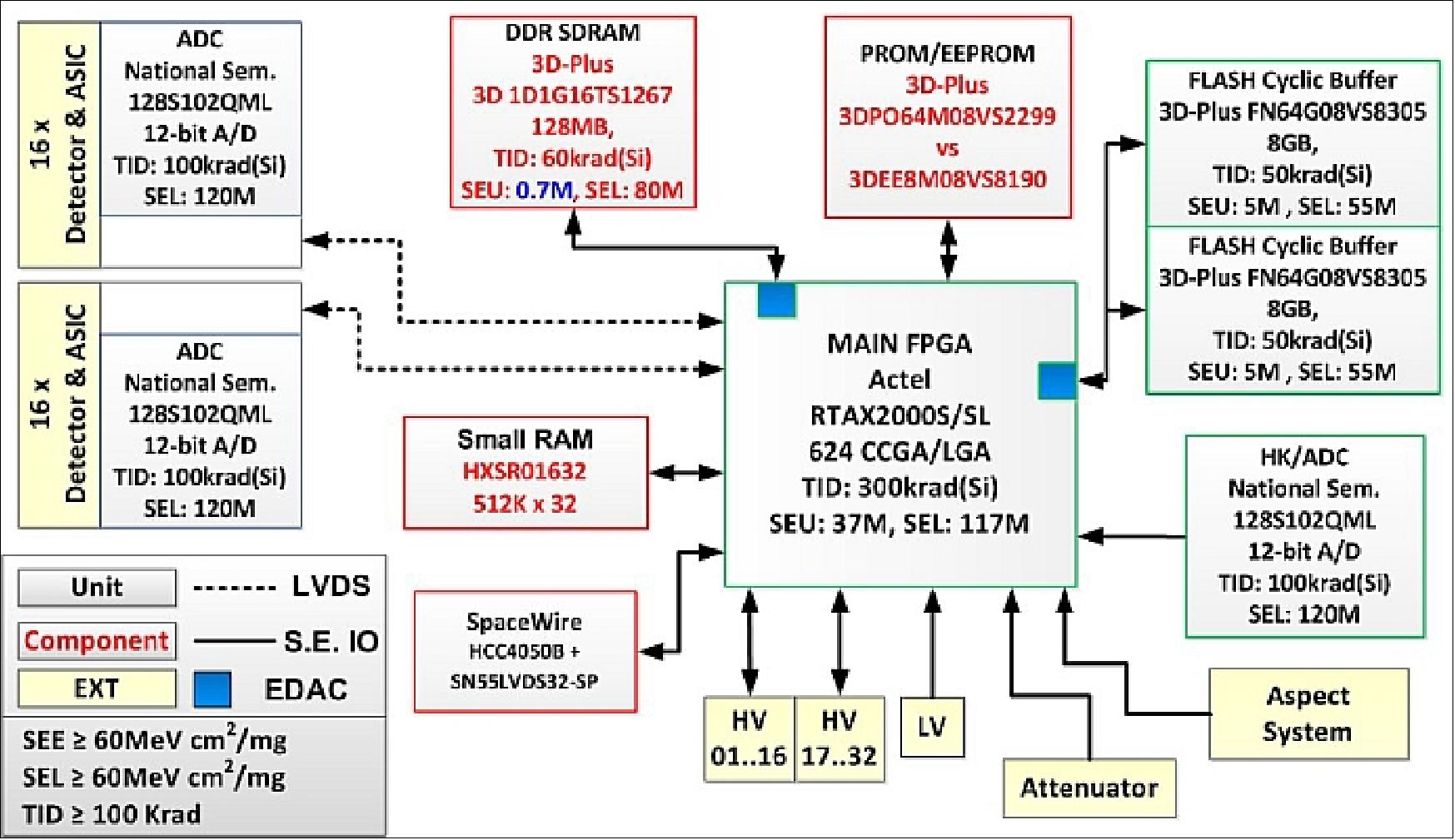
SolOHI (Solar Orbiter Heliospheric Imager)
SolOHI is an NRL (Naval Research Laboratory), Washington D.C. optical telescope which was chosen by ESA in January 2012 to fly on the SolO mission. SolOHI's revolutionary measurements will allow scientists to identify CME's (Coronal Mass Ejections), which are space weather events. These solar eruptions can travel from 120 to 3,500 km/s and have masses greater than a few billion tons. CMEs can affect electromagnetic fields on Earth impacting power lines, satellite communications, and cell phone service. 96)
SolOHI is an evolution of the HI (Heliospheric Imager) developed for the STEREO SECCHI instrument, but with a new light-weight camera system designed to handle the increased radiation levels that are expected to be seen. The FOV (Field of View) of SolOHI is 40º, twice that of SECCHI/HI-1. At perihelion it will have resolution comparable to LASCO/C2 with the C3 FOV is a visible-light telescope that images the light scattered from free electrons in the solar wind plasma. Thus it observes solar wind structures – streamers or plasma sheets, CMEs, density fluctuations, comets, etc. 97) 98)
SolOHI's detector is a 4 k x 4 k APS (Advanced Pixel Sensor) using CMOS technology. This will be the first time such a large format APS detector is flown. The instrument will make high-resolution images of the corona and solar wind, including CMEs, to determine how they propagate and interact with the background solar wind. With its large FOV, SolOHI will be able to connect the remote sensing observations of the corona to the plasma being measured in-situ at the spacecraft.
The instrument will incorporate a mosaic of SRI-designed complementary metal-oxide semiconductor (CMOS) image sensors. The full-flight SolOHI focal plane will use four of these 2k x 2k side-buttable imagers providing a 4 k x 4 k, or 16 megapixel, format. This will be the first flight for such a large-format CMOS detector. 99)
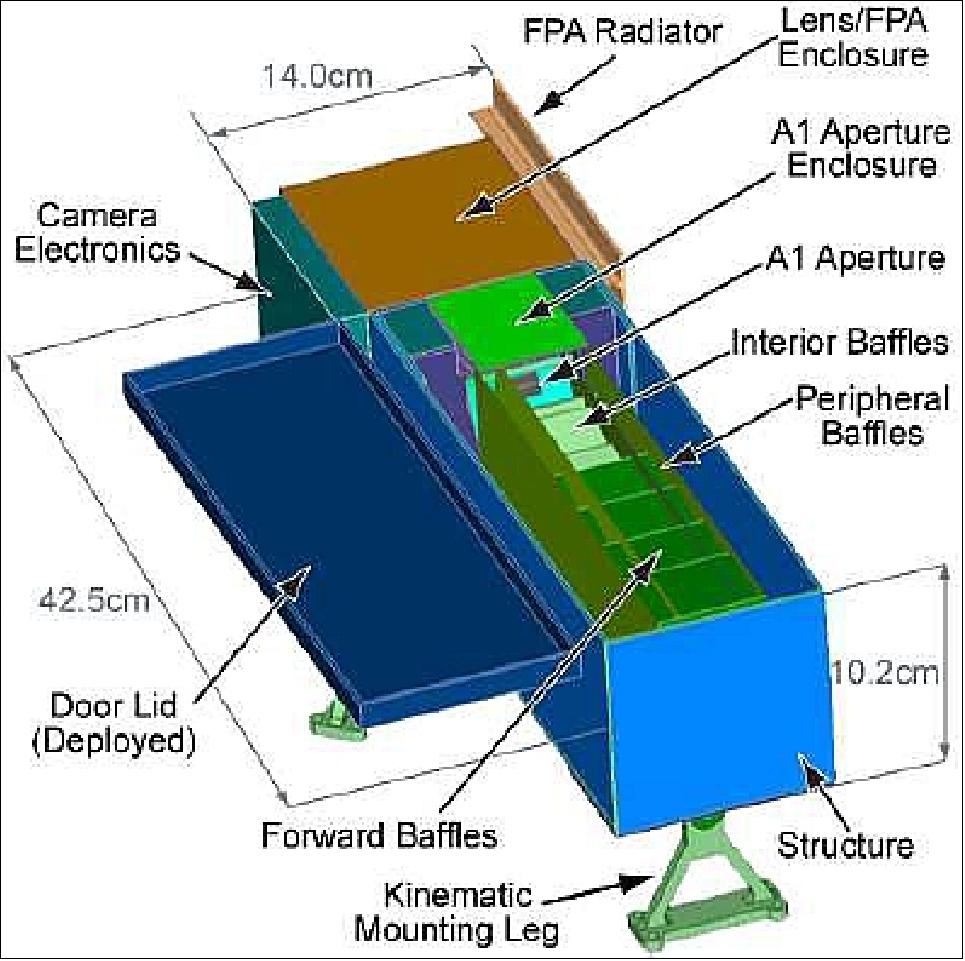
Spacecraft subsystem | Science instrument element | SolOHI design with reserves |
Mechanical | - Instrument mass, instrument electronics mass | 4.48 kg, 4.13 kg |
Power | - Electronics power | 10 W |
C&DH/RF | - Data interfaces | SpaceWire |
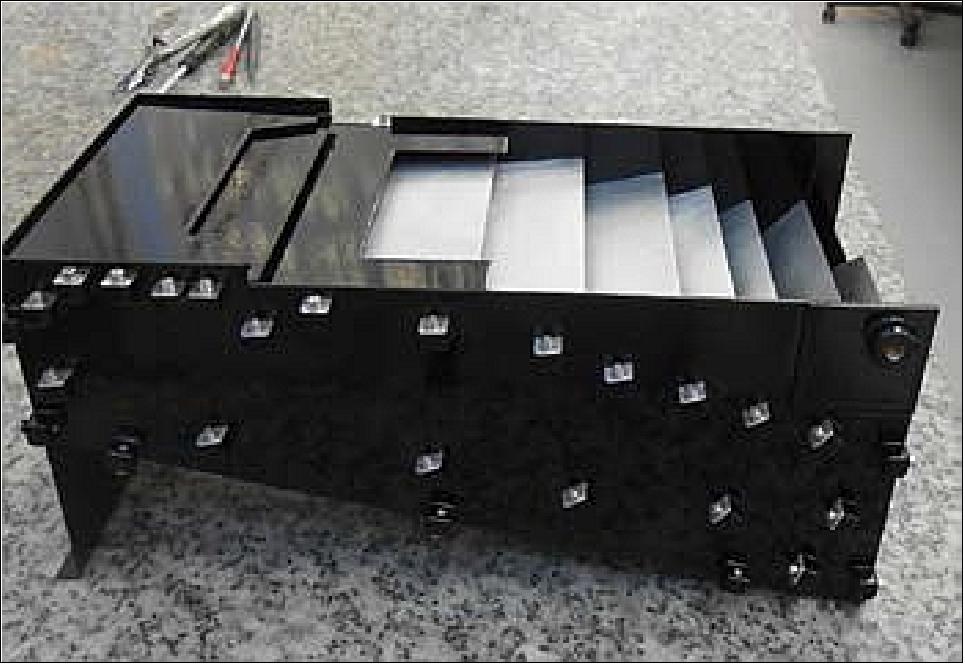
SolOHI is funded by NASA under its Living with a Star Program, which is designed to understand how and why the sun varies, how planetary systems respond and the effect on human space and Earth activities. NASA's Goddard Space Flight Center in Greenbelt, MD, manages the program for the agency's Heliophysics Division of NASA's Science Mission Directorate.
Heliospheric In-situ Instrument Package
SWA (Solar Wind Plasma Analyzer)
The SWA investigation is a major international hardware collaboration, led by UCL/MSSL (PI: Christopher J. Owen). In addition to the overall leadership of the suite, UCL/MSSL will provide the bulk of the hardware for the EAS (Electron Analyzer System), one of the 3 sensor systems within the suite. The PAS (Proton-Alpha Sensor) and the HIS (Heavy Ion Sensor) are led by partners in France and the USA, respectively. The central data processing unit, to be built in Italy, serves all 3 sensors and completes the suite. 100) 101)
The prime scientific objectives of SWA are:
- To provide observational constraints on kinetic plasma properties for a fundamental and detailed theoretical treatment of all aspects of coronal heating
- To investigate charge- and mass-dependent fractionation processes of the solar wind acceleration process in the inner corona
- To correlate comprehensive in-situ plasma analysis and compositional tracer diagnostics with spaceborne and ground-based optical observations of individual stream elements.
In addition, the SWA instrument will enable the investigation of:
- 3He and “unusual” charge states in CME-related flows
- The interaction of solar wind ions on dust grains in the heliocentric distance range associated with the “inner source.” Freshly produced pick-up ions from this inner source are specially suited as test particles for studying the dynamics of incorporation of these particles into the solar wind or their further re-energization.
Instrument concept: SWA is a compromise between sensitivity, mass/charge- and mass- and time resolution parameters - due to the limited resources allocated for the instrument. SWA has to cover a large dynamic range in ion fluxes. Since there is an enormous difference between the proton fluxes at perihelion (typically 1014 m-2 s-1) and the fluxes of relevant minor ion tracers at 1 AU (e.g. Fe10+ at typically 108 m-2 s-1 etc.), it is suggested to implement three different sensors:
• PAS (Proton/α-particle Sensor). The objective is to investigate the velocity distribution of the major ionic species at a time resolution equivalent to the ambient proton cyclotron frequency. The sensor is sun pointing. SWA/PAS consists of an electrostatic analyzer with an ion steering (IS) system at the aperture. It is designed to measure the full 3D velocity distribution functions of the major solar wind species, protons and α-particles, in the energy range ≤ 0.2–20 keV/q. 102)
The SWA/PAS instrument was built by IRAP (Institut de Recherche en Astrophysique et Planétologie), Toulouse, France with with technical and hardware contributions from UCL/MSSL and Charles University, Prague. Key challenges: These include development of a sensor heat shield to deal with the Sun-facing thermal environment, engineering the structure of the sensor to adequately protect against the 150 krad radiation environment over mission lifetime, and to design the sensor to handle the large dynamic range of the expected ion fluxes.
• EAS (Electron Analyzer System). The instrument consists of two (three optional if resources allow) sensors to cover nearly 4π steradian of viewing space and to allow the determination of the primary moments of the electron velocity distribution with high temporal resolution.
• HIS (Heavy Ion Sensor). The instrument of SwRI (Southwest Research Institute) allows the independent determination of the major charge states of oxygen and iron (up to 9) and a coarse mapping of the three-dimensional velocity distribution of some prominent minor species. Also, pick-up ions of various origins, such as weakly-ionized species (C+, N+, Ne+, Mg+, Si+, etc.), should be measured. The sensor is sun pointing.
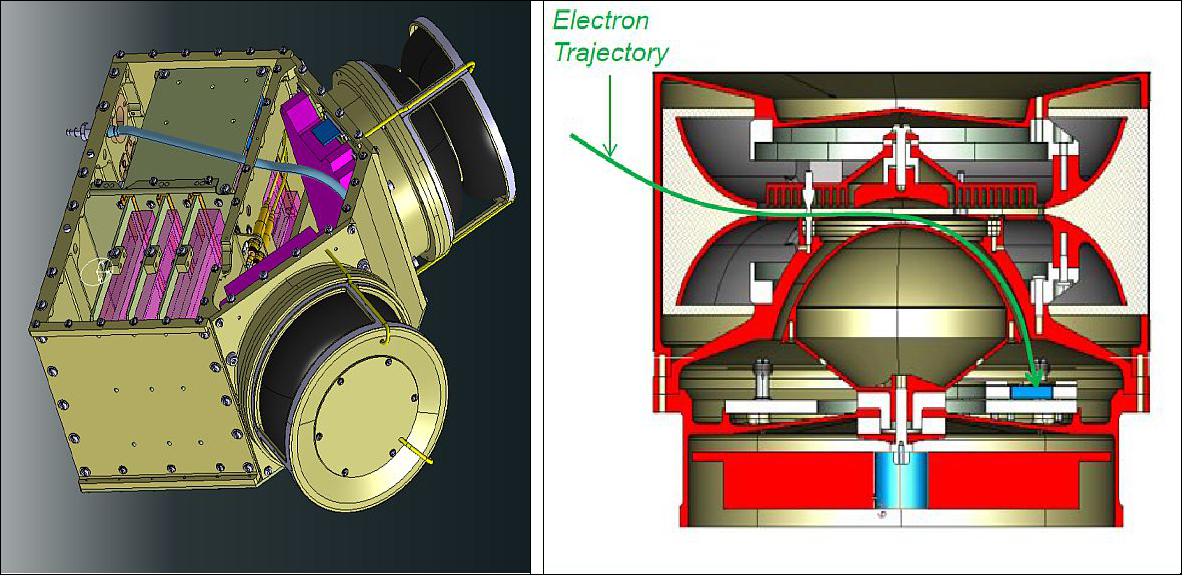
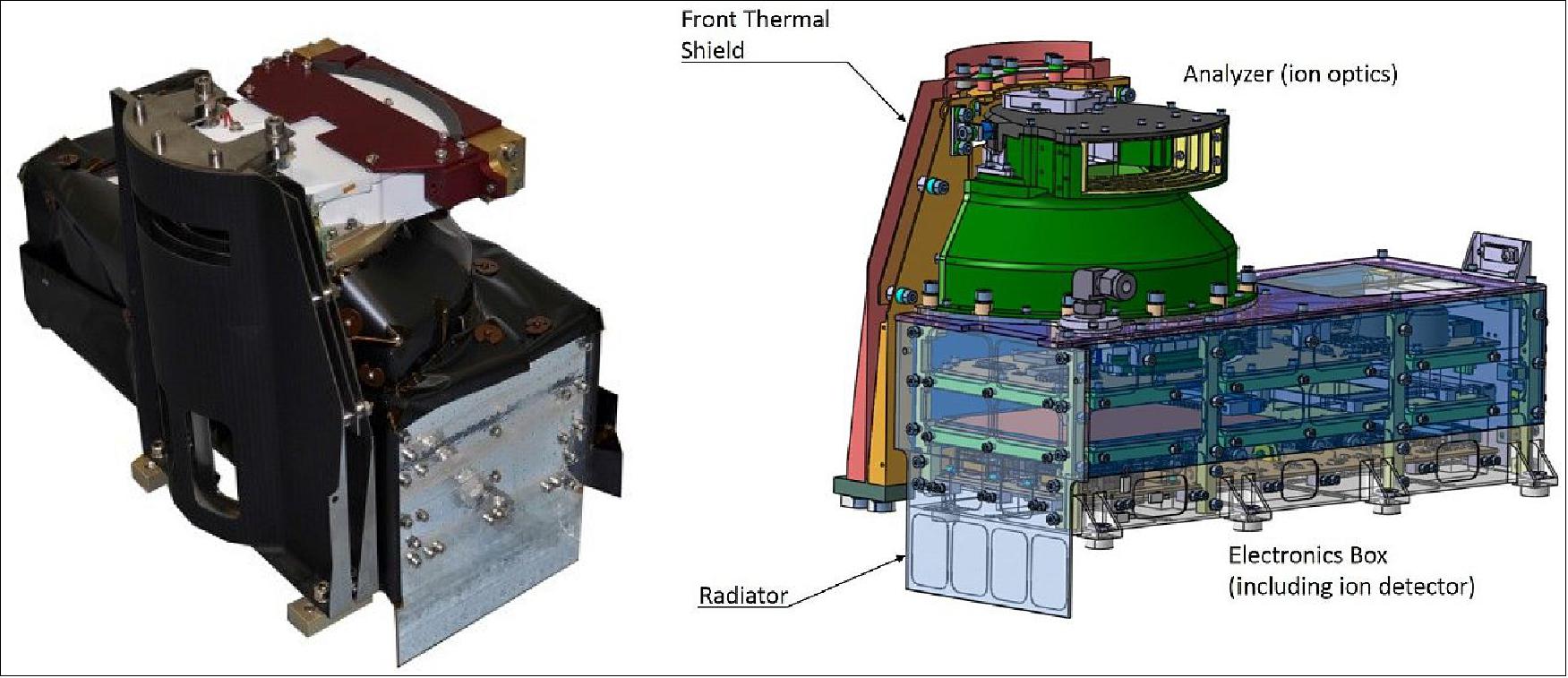
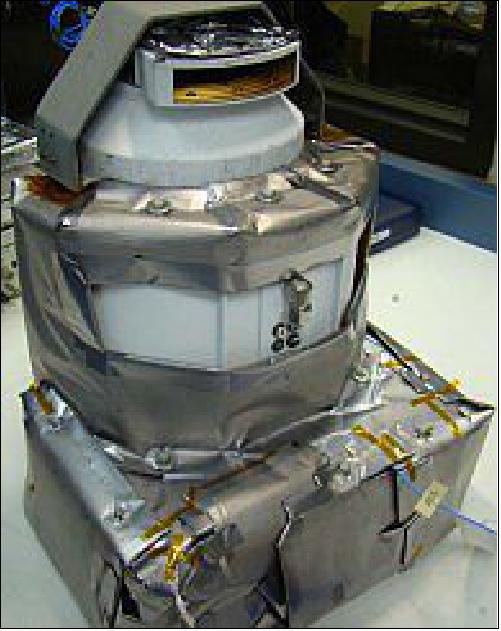
RPW (Radio & Plasma Wave Analyzer)
The prime objective of RPW is to provide measurements of both the electric field and magnetic field in a broad frequency band (typically from a fraction of a Hertz up to several tens of MHz) covering characteristic frequencies in the solar corona and interplanetary medium. The measurements of both electrostatic and electromagnetic waves provide different diagnostics:
- Electrostatic waves provide in-situ information in the vicinity of the spacecraft
- Electromagnetic waves provide extensive remote-sensing of energetic phenomena in the solar corona and interplanetary medium.
In addition, the RPW instrument on Solar Orbiter will enable the investigation of:
- Waves and turbulence that occur much closer to the sun than previously measured
- The north-south symmetry of the radio radiation in the solar corona using, for the first time, viewing angles from well out of the ecliptic plane.
Instrument concept: RPW comprises two main sub-systems: PWS (Plasma Waves System) covering in-situ measurements, and the RAD (Radio Astronomy Detector) for remote-sensing.
Principal Investigator of RPA: Milan Maksimovic, LESIA, Observatoire de Paris, France. Collaborating countries (hardware): France, Sweden, Czech Republic, Austria.
MAG (Magnetometer)
MAG will provide in-situ measurements of the heliospheric magnetic field. Scientific topics to be addressed by MAG include the way the Sun’s magnetic field links into space and evolves over the solar cycle; how particles are accelerated and propagate around the solar system, including to the Earth; how the corona and solar wind are heated and accelerated. The magnetometer instrument will comprise two sensors, both in shadow and mounted on a boom behind the spacecraft body.
The prime objective of MAG is to provide vector measurements of the solar wind magnetic field with high resolution (better than 1 nT) at sub-second sampling. The MAG instrument will enable the investigation of: 103)
- The link between coronal structures and their signatures in the solar wind
- Kinetic effects in the solar wind plasma
- Large-scale structures in the solar wind, e.g., coronal mass ejections
- MHD waves and turbulence.
The reference MAG instrument consists of dual 3-axis fluxgate sensors mounted on a deployable boom, which is positioned in the shadow of the Orbiter body. One sensor is at the end of the boom and the other at an intermediate distance from the spacecraft body. It measures fields in several gain-ranges, which are automatically selected by the DPU according to the in-situ magnetic field strength. This configuration allows compensation for spacecraft generated stray fields.
Principal Investigator: Tim Horbury, ICSTM, London, United Kingdom. Collaborating countries (hardware): United Kingdom.
EPD (Energetic Particle Detector)
The EPD suite contains five different sensors to measure the composition, timing, and distribution functions of suprathermal and energetic particles, such as, electrons and protons. Principal Investigator: Javier Rodríguez-Pacheco, University of Alcala, Spain. Collaborating countries (hardware): Spain, Germany, USA, ESA.
The prime objectives of EPD are:
- To determine in-situ the generation, storage, release and propagation of different species of solar energetic particles in the inner heliosphere
- To identify the links between magnetic activity and acceleration on the sun of energetic particles, by virtue of combined remote-sensing of their source regions and in-situ measurements of their properties
- To characterize gradual (typically CME-related) and impulsive (typically flare-related) particle events and trace their spatial and temporal evolution near the sun
- To measure energetic pick-up particles originating from the interaction of the solar wind with near-sun dust.
The EPD will determine chemical and charge composition and energy spectra of ions in a wide energy range, from about the typical solar wind energies of a few keV to several 100 MeV/nucleon for protons and heavy ions. Electrons should be measured from 10 keV to 10 MeV. The combination of electrostatic E/Q-analysis with time-of-flight E/M-determination and subsequent direct energy measurement in a solid state detector has been employed in many EPDs in the past and is also a possible design option for the Solar Orbiter.
The EPD suite consists of five sensors measuring electrons, protons, and ions from helium to iron, and operating at partly overlapping energy ranges from 2 keV up to 200 MeV/n. The EPD sensors and a common data processing unit with low voltage power supply are: 104)
1) STEIN (SupraThermal Electrons, Ions, and Neutrals)
2) SIS (SupraThermal Ion Spectrograph)
3) EPT (Electron Proton Telescope)
4) LET (Low Energy Telescope)
5) HET (High Energy Telescope)
6) ICU (Instrument Control Unit).
The EPD sensors share the ICU (Instrument Control Unit) that is composed by the Common Data Processing Unit and the Low Voltage Power Supply (CDPU/LVPS), which is the sole power and data interface of EPD to the spacecraft. 105)
• The Institute for Experimental and Applied Physics (IEAP) of the Christian-Albrechts-University (CAU) in Kiel, Germany, is developing four sensors for the EPD (Energetic Particle Detector): STEIN, EPT, HET, and SIS. The development and manufacturing of STEIN, EPT, and HET is funded through DLR (German Space Agency), and SIS is funded by ESA as a facility instrument.
• The AIP (Astrophysikalisches Institut Potsdam) contributes to the development of two of these sensors: EPT (Electron Proton Telescope) and HET (High Energy Telescope).
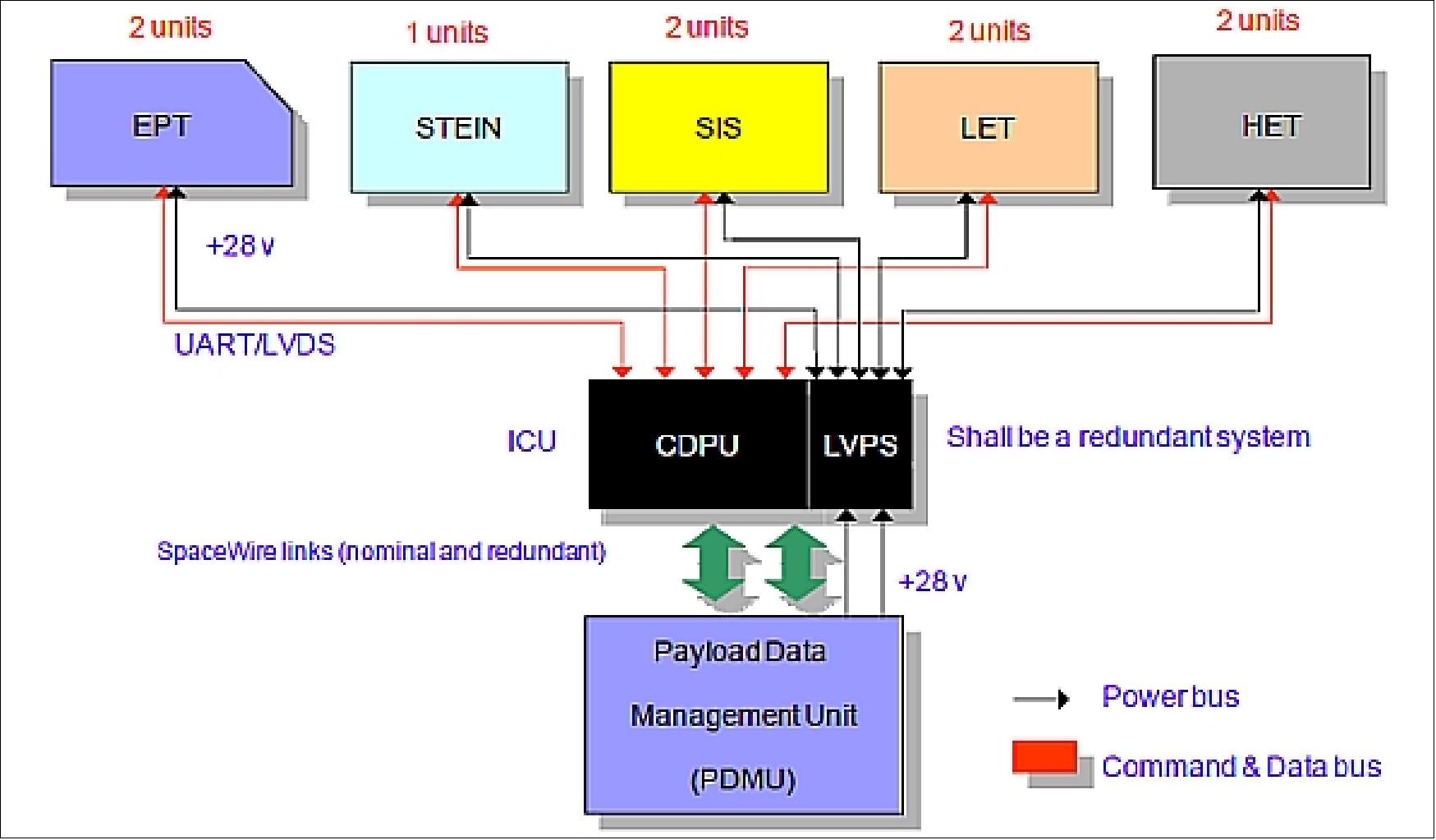
STEIN consists of a single unit having two view cones in opposite directions. SIS consists of two sensor heads with roughly opposite (160°) view directions sharing a common electronics box. EPT -HET has multiple view cones sharing a common electronics box. There are two identical EPT-HET units. LET has multiple view cones and consists of two separate identical units.
EPT consists of two dual double-ended telescopes: EPT1 points in the ecliptic plane along the Parker spiral in both the solar and anti-solar directions; EPT2 points 45º out of the ecliptic plane. Each sensor unit consists of a dual double-ended magnet/foil solid state detector particle telescope. One of the major scientific goals of SolO will be the investigation of particle acceleration and propagation at the Sun. Electrons, as measured by the EPT, play a crucial role in the study of energetic processes on the Sun as they provide a direct link to the sites of particle acceleration. Due to the high sensitivity of the EPT it will measure small energetic particle events and even nano flare particles (Ref. 95).
HET covers the high-energy particle range for protons (up to 100 MeV) and heavier ions, thus providing information on the largest solar energetic particle events, which can produce high energy, damaging interplanetary radiation levels. HET has two oppositely directed field of views with 50º full angle and resolves 3Helium and multiple heavy ion species. HET's large collecting power allows fast cadence for high-energy heavy ions. During the study phase, the possibility of obtaining limited information on the neutron flux intensity from HET will be investigated.
HET consists of two sensor double-ended heads, one pointing sun/anti-sunward, the other out of the ecliptic. Thus, HET has a total of four viewing directions. Both HET sensors are identical and consist of a double-ended set of solid state detectors and a high-density calorimeter scintillator. Current trades still involve BGO and GSO as scintillator materials. 106)
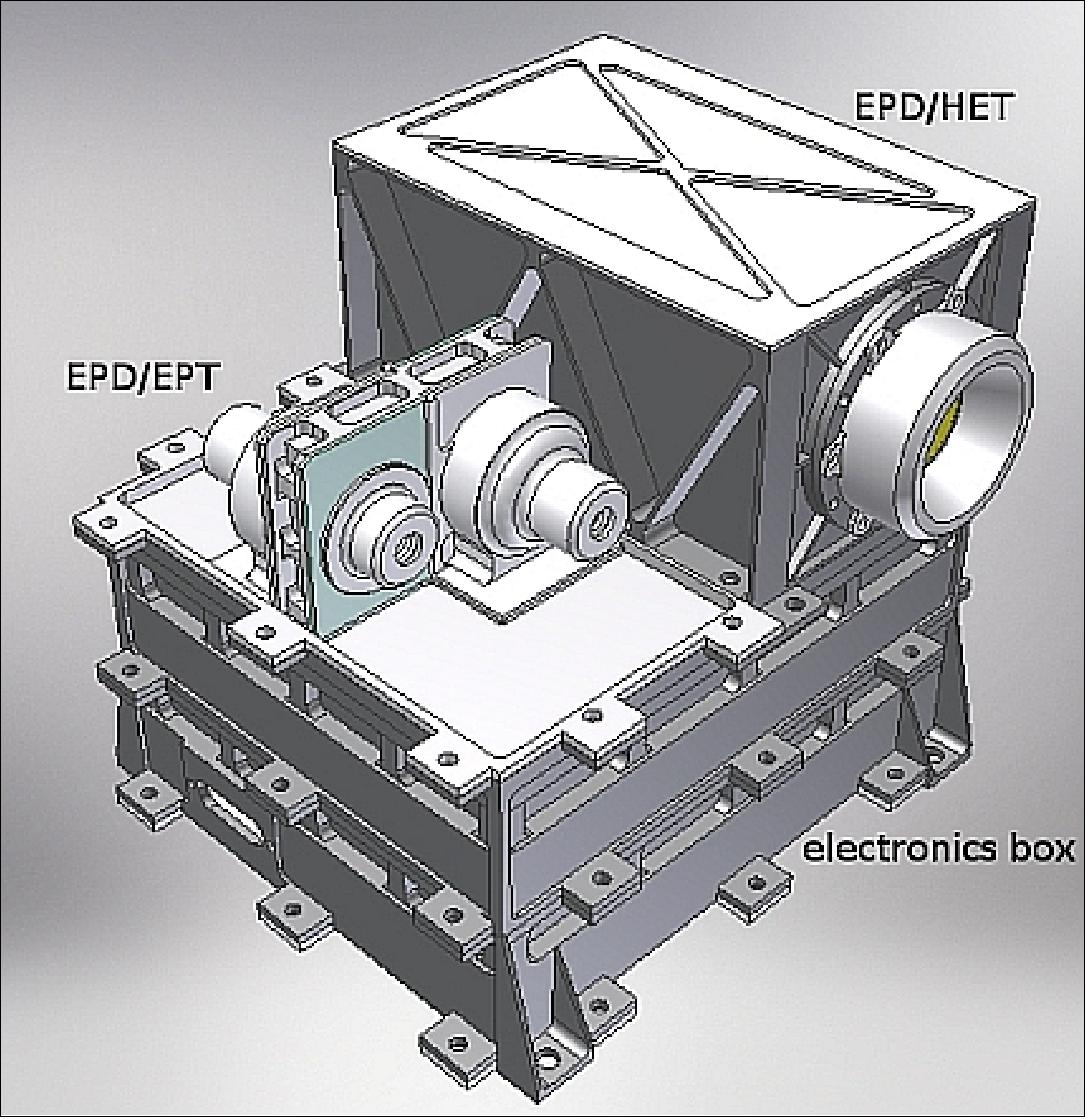
LET (Low Energy Telescope) is designed to identify elements from H to Ni with excellent element and energy resolution over the range from ~1.5 to ~60 MeV/nucleon where SEP spectral breaks typically occur. It will also separate 3He from 4He down to levels of ~1% and resolve Ne and Mg isotopes. In addition, the broad dynamic range will provide measurements of trans-Fe elements with 30 < Z < 83, which are often enriched by factor of ~100 in impulsive SEP events. LET accomplishes these measurements using the well-known E-E method of particle detection in six small stacks of silicon detectors. Using the energy-loss patterns in successive detector layers, the charge, mass, and kinetic energy of species from H to Ni can be uniquely identified onboard at event rates up to ~10 kHz. The LET design has heritage from SOHO/ERNE, which has more than 14 years of successful operation in space. 107)
The basic structure of LET is shown in Figure 129. Two circular front detectors, D1 and D2, define the view cones of the three telescopes. The thickness of the D1 detector is 20 µm, while D2 is 80 µm thick. The thickness of D1 is the main factor determining the lower limit of the operational energy range of LET and it also determines the proton upper energy range due to the decreasing signal with increasing energy. For particle identification, it is required that the particle penetrates the thin foil (e.g., 8 µm polyimide with aluminum coating) above D1, the detector D1 itself, and gives a signal (energy loss of ~150 keV) from D2. Below D2, the three silicon detectors, D3, D4, and D5 have sufficient thickness to stop all particles with energies below the defined higher limit of the operational energy range. At the bottom of each telescope, a detector (AC) is used in anti-coincidence with the other detectors to reject penetrating particles. The front detectors D1 and D2 are both divided in three active areas, a center area and a surrounding annular area with two segments. This division gives angular resolution within the 40º view cone of the three telescopes. More importantly, the field of view for proton and helium measurements in each of the three directions can be dynamically limited to a cone with a full opening angle of 14º, while simultaneously maintaining the full opening angle for heavy ion measurements. This feature allows high-sensitivity measurements of even small solar particle events, while ensuring unsaturated operation during very high flux conditions of large SEP (Solar Energetic Proton) events. The total and center area geometric factors are 0.21 cm2sr and 0.0024 cm2 sr, respectively, giving total geometry factors of 1.26 and 0.014 cm2 sr for six telescopes. The collecting power of the first detector alone, determined by the active surface of D1 and the edge of the collimator structure, is 1.68 cm2 sr.
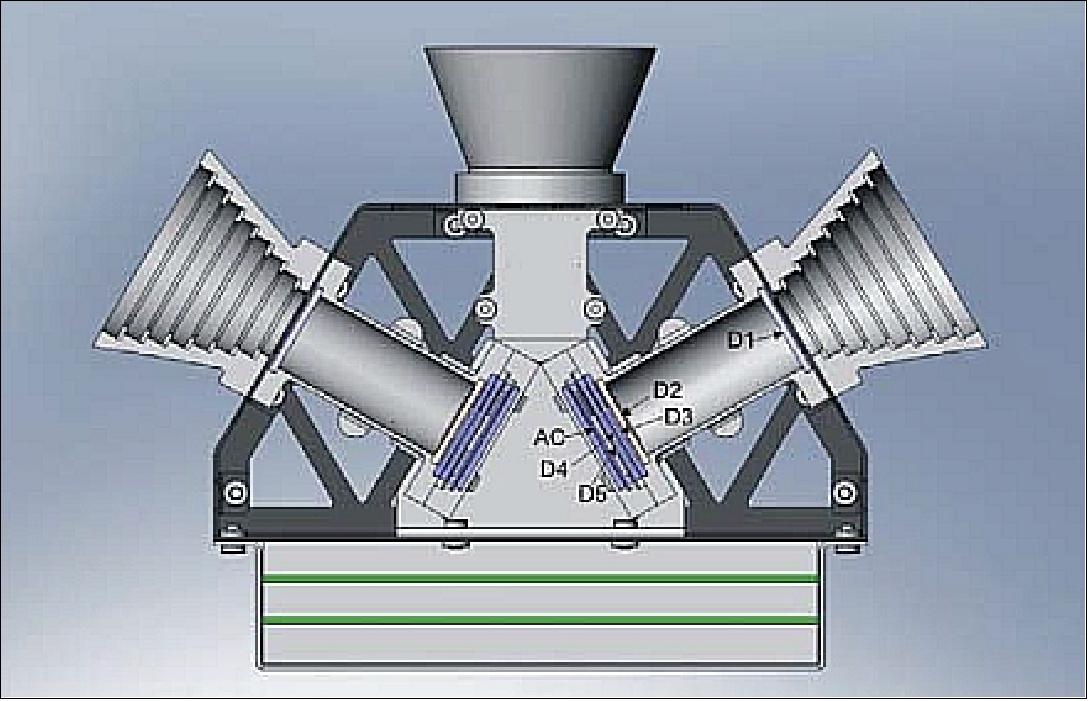
The analyzed data are transferred to the ICU once per second, which allows implementation of a burst mode with 1 second cadence. However, the basic time resolution of LET is 10 s. Proton, 3He, and 4He energy spectra and samples of PHA data are accumulated by the ICU software with this cadence. A longer integration time of 100 s is used for the spectra of heavier ions.
SIS (SupraThermal Ion Spectrograph): SIS identifies particles by time-of-flight (TOF) mass spectrometry. It covers the energy range from ~0.008 MeV/nucleon to 10 MeV/nucleon for He to Fe. SIS consists of two units, each with one particle telescope (sunward and antisunward) located near a single electronics box with power and data interface to the ICU. Particles are detected when they pass through the entrance foil and trigger one of the solid state detectors (SSDs) in the array in the back. Secondary electrons emitted when the ion passes through the entrance-, mid-, and detector foils are accelerated to ~1 kV and directed via isochronous mirrors onto MCPs (Micro-Channel Plates). Fast signals from the MCPs provide Start-1, Start-2, and STOP signals that are analyzed to obtain the time-of-flight measurements for the ions. The SSD measures the residual energy of the ion. By combining the two parameters, and the known flight path of the telescope, the mass of the ion can be identified individually. SIS's high-mass resolution (m/sm ~ 50) allows the project to identify 3He/4He ratios down to <1 %. SIS is now being developed under our European lead in collaboration with Spain's SENER and the Johns Hopkins University Applied Physics Laboratory(JHU/APL) in Laurel, MD, USA.
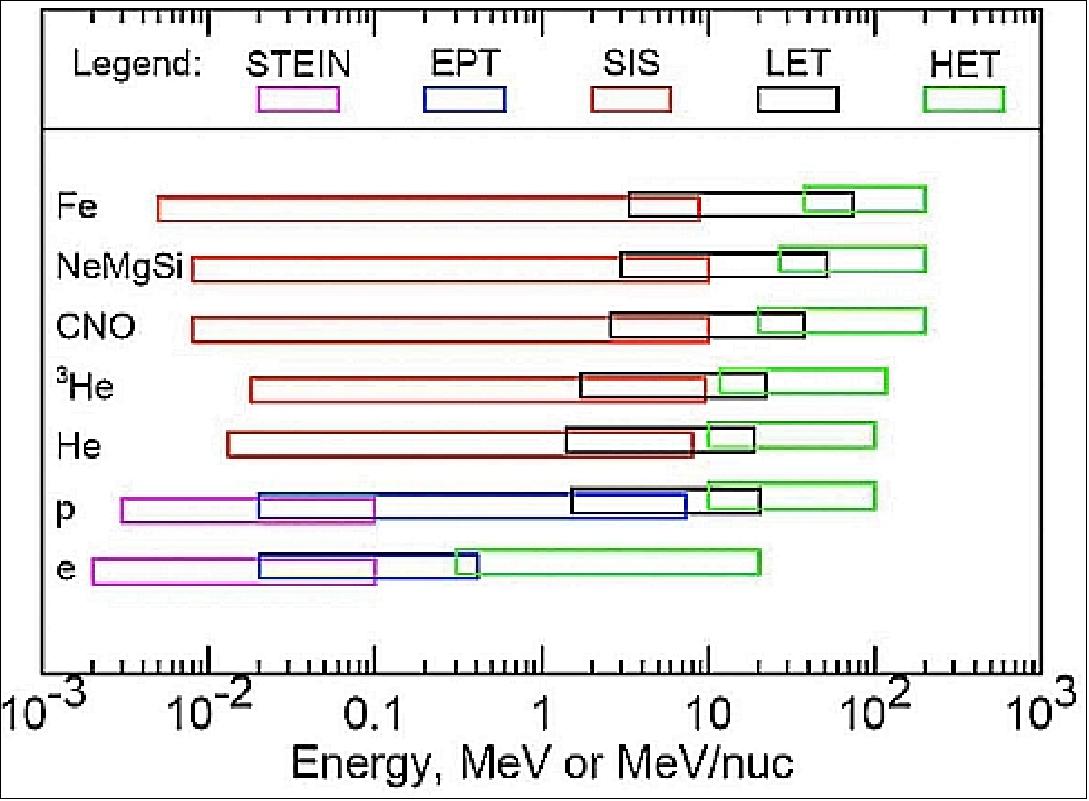
ICU (Instrument Control Unit): ICU is composed by the CDPU (Common Data Processing Unit) and the LVPS (Low Voltage Power Supply). It provides a single point digital interface to the spacecraft and to all EPD sensors (STEIN, HET-EPT, LET and SIS). It also shares information with the STIX, MAG and SWA investigation to allow synchronized high data rate burst-mode operations following on-board identification of predefined triggering events in the EPD data. The ICU is designed to manage sensors control and monitoring, the sensors timing clock, and sensor data collection, compression, and packetization for telemetry. ICU is also responsible of the S/C telecommand reception and delivery, if necessary, to the sensors.
The ICU box is mounted inside the spacecraft body. The box dimensions are 186 mm x 152 mm x 100 mm. The CDPU contains a LEON 2 microprocessor, RAM, EEPROM and PROM memories, two hot redundant SpaceWire interfaces, and six identical serial sensor interfaces. The CDPU PROM contains boot code that can load flight code from either the EEPROM or from the spacecraft interface via telecommand. The EEPROM contains TBD copies of the flight code. The RAM (up to 1 Gbit) is used for code, variables, and buffers. With 1 Gbit memory, at 3.100 Mbit/s download rate, up to four days of data can be stored in this memory. Most of the CDPU RAM is used for a burst memory to save high time resolution interval snapshots from STEIN, HET-EPT, LET and SIS, which are then played out slowly via telemetry. In case of power on/off cycling, the CDPU will reset and resume operations from a defined mode.
The LVPS board is responsible of filtering, monitoring and switching the spacecraft primary power, ranging from 26 -29 V (in accordance with EID-A [AD 1] limits), to the sensors. It also provides the power supply to both CDPUs, nominal and redundant. In the latter case, +3.3 V and +1.5 V are provided. The LVPS will be designed to operate with nominal performance within a power bus voltage of 28 V with variations from 26 -29 V. The ICU includes following protection mechanisms for the sensors:
• Under-voltage protection: The LCLs (Low Capacitive Limit) switches, responsible of switching on/off the sensors, are only enabled if the input power bus voltage is above certain level and are automatically switched off if this power bus voltage drops below it.
• Short-circuit protection: If a short-circuit is produced in the primary power of any sensor, the corresponding LCL limits its current during some milliseconds. After that, this LCL is switched off.
A Short Presentation of the Two Solar Missions: Parker SP (Parker Solar Probe) of NASA and Solar Orbiter of ESA
• May 16, 2018: Two upcoming missions will soon take us closer to the Sun than we’ve ever been before, providing our best chance yet at uncovering the complexities of solar activity in our own solar system and shedding light on the very nature of space and stars throughout the universe. 108)
- Together, NASA’s Parker Solar Probe and ESA’s (the European Space Agency) Solar Orbiter may resolve decades-old questions about the inner workings of our nearest star. Their comprehensive, up-close study of the Sun has important implications for how we live and explore: Energy from the Sun powers life on Earth, but it also triggers space weather events that can pose hazard to technology we increasingly depend upon. Such space weather can disrupt radio communications, affect satellites and human spaceflight, and — at its worst — interfere with power grids. A better understanding of the fundamental processes at the Sun driving these events could improve predictions of when they’ll occur and how their effects may be felt on Earth.
- “Our goal is to understand how the Sun works and how it affects the space environment to the point of predictability,” said Chris St. Cyr, Solar Orbiter project scientist at NASA’s Goddard Space Flight Center in Greenbelt, Maryland. “This is really a curiosity-driven science.”
- The Parker Solar Probe was launched on 12 August 2018, and Solar Orbiter is scheduled to follow in 2020. These missions were developed independently, but their coordinated science objectives are no coincidence: Parker Solar Probe and Solar Orbiter are natural teammates.
- Both missions will take a closer look at the Sun's dynamic outer atmosphere, called the corona. From Earth, the corona is visible only during total solar eclipses, when the Moon blocks the Sun's most intense light and reveals the outer atmosphere’s wispy, pearly-white structure. But the corona isn’t as delicate as it looks during a total solar eclipse — much of the corona’s behavior is unpredictable and not well understood.
- The corona’s charged gases are driven by a set of laws of physics that are rarely involved with our normal experience on Earth. Teasing out the details of what causes the charged particles and magnetic fields to dance and twist as they do can help us understand two outstanding mysteries: what makes the corona so much hotter than the solar surface, and what drives the constant outpouring of solar material, the solar wind, to such high speeds.
- We can see that corona from afar, and even measure what the solar wind looks like as it passes by Earth — but that’s like measuring a calm river miles downstream from a waterfall and trying to understand the current’s source. Only recently have we had the technology capable of withstanding the heat and radiation near the Sun, so for the first time, we’re going close to the source.
- “Parker Solar Probe and Solar Orbiter employ different sorts of technology, but — as missions — they’ll be complementary,” said Eric Christian, a research scientist on the Parker Solar Probe mission at NASA Goddard. “They’ll be taking pictures of the Sun’s corona at the same time, and they’ll be seeing some of the same structures — what's happening at the poles of the Sun and what those same structures look like at the equator.”
- Parker Solar Probe will traverse entirely new territory as it gets closer to the Sun than any spacecraft has come before — as close as 3.8 million miles from the solar surface. If Earth were scaled down to sit at one end of a football field, and the Sun at the other, the mission would make it to the 4-yard line. The current record holder, Helios B, a solar mission of the late 1970s, made it only to the 29-yard line.
- From that vantage point, Parker Solar Probe’s four suites of scientific instruments are designed to image the solar wind and study magnetic fields, plasma and energetic particles — clarifying the true anatomy of the Sun’s outer atmosphere. This information will shed light on the so-called coronal heating problem. This refers to the counterintuitive reality that, while temperatures in the corona can spike upwards of a few million degrees Celsius, the underlying solar surface, the photosphere, hovers around just 6,000ºC. To fully appreciate the oddity of this temperature difference, imagine walking away from a campfire and feeling the air around you get much, much hotter.
- Solar Orbiter will come within 26 million miles of the Sun — that would put it within the 27-yard line on that metaphorical football field. It will be in a highly tilted orbit that can provide our first-ever direct images of the Sun’s poles — parts of the Sun that we don’t yet understand well, and which may hold the key to understanding what drives our star’s constant activity and eruptions.
- Both Parker Solar Probe and Solar Orbiter will study the Sun’s most pervasive influence on the solar system: the solar wind. The Sun constantly exhales a stream of magnetized gas that fills the inner solar system, called solar wind. This solar wind interacts with magnetic fields, atmospheres, or even surfaces of worlds throughout the solar system. On Earth, this interaction can spark auroras and sometimes disrupt communications systems and power grids.
- Data from previous missions have led scientists to believe the corona contributes to the processes that accelerate particles, driving the solar wind’s incredible speeds — which triple as it leaves the Sun and passes through the corona. Right now, the solar wind travels some 92 million miles by the time it reaches the spacecraft that measure it — plenty of time for this stream of charged gases to intermix with other particles traveling through space and lose some of its defining features. Parker Solar Probe will catch the solar wind just as it forms and leaves the corona, sending back to Earth some of the most pristine measurements of solar wind ever recorded. Solar Orbiter’s perspective, which will provide a good look at the Sun’s poles, will complement Parker Solar Probe’s study of the solar wind, because it allows scientists to see how the structure and behavior of the solar wind varies at different latitudes.
- Solar Orbiter will also make use of its unique orbit to better understand the Sun’s magnetic fields; some of the Sun’s most interesting magnetic activity is concentrated at the poles. But because Earth orbits on a plane more or less in line with the solar equator, we don’t typically get a good view of the poles from afar. It’s a bit like trying to see the summit of Mount Everest from the base of the mountain.
- That view of the poles will also go a long way toward understanding the overall nature of the Sun’s magnetic field, which is lively and extensive, stretching far beyond the orbit of Neptune. The Sun’s magnetic field is so far-reaching largely because of the solar wind: As the solar wind streams outward, it carries the Sun’s magnetic field with it, creating a vast bubble, called the heliosphere. Within the heliosphere, the solar wind determines the very nature of planetary atmospheres. The heliosphere’s boundaries are shaped by how the Sun interacts with interstellar space. Since Voyager 1’s passage through the heliopause in 2012, we know these boundaries dramatically protect the inner solar system from incoming galactic radiation.
- It’s not yet clear how exactly the Sun’s magnetic field is generated or structured deep inside the Sun — though we do know intense magnetic fields around the poles drives variability on the Sun, causing solar flares and coronal mass ejections. Solar Orbiter will hover over roughly the same region of the solar atmosphere for several days at a time while scientists watch tension build up and release around the poles. Those observations may lead to better awareness of the physical processes that ultimately generate the Sun’s magnetic field.
- Together, Parker Solar Probe and Solar Orbiter will refine our knowledge of the Sun and heliosphere. Along the way, it’s likely these missions will pose even more questions than they answer — a problem scientists are very much looking forward to.
- "There are questions that have been bugging us for a long time," said Adam Szabo, mission scientist for Parker Solar Probe at NASA Goddard. "We are trying to decipher what happens near the Sun, and the obvious solution is to just go there. We cannot wait — not just me, but the whole community."
References
1) “Dark and bright: ESA chooses next two science missions,” ESA, Oct. 4, 2011, URL: http://www.esa.int/esaCP/SEMOZ59U7TG_index_0.html
2) Richard G. Marsden, Daniel Müller, “Solar Orbiter - Exploring the Sun-heliosphere connection,” Definition Study Report, ESA/SRE (2011) 14, July 2011, URL: http://sci.esa.int/science-e/www/object/doc.cfm?fobjectid=48982
3) C. Erd, “ESA Science Missions: Plans of the Cosmic Vision program,” ESA/ESTEC, March 15, 2011, URL: https://escies.org/download/webDocumentFile?id=49230
4) Solar Orbiter Science Working Team, “Solar Orbiter- Exploring the Sun-heliosphereconnection, Definition Study Report” ESA/SRE(2011), July 14, 2011, URL: http://www.solarorbiter.org/documents/reports/Solar_Orbiter-RedBook.pdf
5) “Space Cooperation: Solar Orbiter Mission,” March 2012, URL: http://www.state.gov/documents/organization/197206.pdf
6) http://sci.esa.int/science-e/www/area/index.cfm?fareaid=45
7) “Solar Orbiter Assessment Phase Final Executive Report,” SCI-A/2005/054/NR, issue 2.0, Dec. 15, 2005, http://sci.esa.int/science-e/www/object/doc.cfm?fobjectid=37623
8) http://www.esa.int/esaSC/120384_index_0_m.html
9) Mark Ayre, “Solar Orbiter: The next ESA mission to the Sun,” Proceedings of the 59th IAC (International Astronautical Congress), Glasgow, Scotland, UK, Sept. 29 to Oct. 3, 2008, IAC-08.A3.6.3
10) http://www.bis.gov.uk/ukspaceagency/missions/solar-orbiter
11) G. Colangelo, O, Pace, R. Marsden, B. Fleck, “Solar Orbiter: A Challenging Mission Design for Near-Sun Observations,” ESA Bulletin, No 104, Nov. 2000, pp. 76-85, URL: http://zeus.nascom.nasa.gov/~bfleck/Preprints/ESA_Bull104.pdf
12) Richard Marsden, “Solar Orbiter Science Management Plan,” ESA, SOL-EST-PL-00880, Issue 2, Revision 2, Feb. 16, 2012, URL: http://sci.esa.int/science-e/www/object/doc.cfm?fobjectid=50621
13) “Solar Orbiter,” ESA, Aug. 13, 2013, URL: http://www.esa.int/Our_Activities/Space_Science/Solar_Orbiter
14) Daniel Müller, O. Chris St. Cyr, “Solar Orbiter - Exploring the Sun-Heliosphere Connection,” Proceedings of SPIE, Vol. 8862, 'Solar Physics and Space Weather Instrumentation V,' San Diego, CA, USA, Aug. 25, 2013, URL: http://www.solarorbiter.org/documents/presentations/overview/Solar_Orbiter_SPIE_Aug2013_dmueller.pdf
15) “ESA contracts Astrium UK to build Solar Orbiter,” ESA, April 26, 2012, URL: http://www.esa.int/esaSC/SEMXQLNW91H_index_0.html
16) Jonathan Amos, “UK industry to build Solar Orbiter satellite,” BBC News, April 26, 2012, URL: http://www.bbc.co.uk/news/science-environment-17743190
17) “From Ariel-1 to Solar Orbiter: Astrium awarded €300 million contract to build the latest mission to study the sun,” April 26, 2012, URL: http://www.bis.gov.uk/ukspaceagency/news-and-events/2012/Apr/astrium-awarded-300-million-contract-to-build-the-latest-mission-to-study-the-sun
18) “Airbus Defence and Space wins €300 million Solar Orbiter contract from ESA,” Airbus DS, April 26, 2012, URL: http://www.space-airbusds.com/en/press_centre/airbus-defence-and-space-wins-eur300-million-solar-orbiter-contract-from-esa.html
19) J. Poncy, F. Jubineau, F. D. Angelo, V. Perotto, J. J. Juliett, “Solar Orbiter - Heat Shield and System Technology,” 58th IAC (International Astronautical Congress), International Space Expo, Hyderabad, India, Sept. 24-28, 2007, IAC-07-A3.2.06
20) A. Lyngvi, N. Rando, L. Gerlach, A. Peacock, “The Solar Orbiter Thermal Design,” Proceedings of the 56th IAC 2005, Fukuoda, Japan, Oct. 17-21, 2005, IAC-05-C2.6.02
21) Solar Orbiter Team, “Solar Orbiter Experiment Interface Document - Part A,” EID-A, Astrium, SOL.EST.RCD.0050, Issue 3, pp. 248, 2012, URL: ftp://ftp.oato.inaf.it/solari/SolarOrbiter/EID-A/12.07.19_EID-A_issue3_SOL.EST.RCD.0050_03_00-1-1.pdf
22) Charles Koeck, Frédéric Faye, Pascal Regnier, S. Boulade, “Solar Orbiter Mission and System Assessment,” Proceedings of the 57th IAC/IAF/IAA (International Astronautical Congress), Valencia, Spain, Oct. 2-6, 2006, IAC-06-A3.2.08
23) Paul Norridge, David Pecover, Joachim Poeckentrup, Stefan Thürey, Wahida Gasti, James Windsor, Michele de Meo, “SpaceWire in Solar Orbiter,” Proceedings of the 5th International SpaceWire Conference 2013, Gothenburg, Sweden, June 10 - 14, 2013, pp: 295-299, URL: http://2013.spacewire-conference.org/downloads/SpW2013ProceedingsFinal.pdf
24) R. Noteborn, L. Stenqvist Hanbury, R. Larsson, S. Veldman, J. Myatt, M. Pigg, M. Yu, A. Prezzavento, J. Touaty, “FDIR and robustness for the Solar OrbiterAOCS,” Proceedings of GNC 2014: 9th International ESA Conference on Guidance, Navigation & Control Systems, Porto, Portugal, June 2-6, 2014, URL: http://www.esa-gnc.eu/papers/porto2014/Session%203b/1015_Noteborn.pdf
25) “Spacecraft,” ESA, Aug. 01, 2013, URL: http://sci.esa.int/solar-orbiter/44168-spacecraft/
26) Solar Orbiter Spacecraft,” ESA, July 30, 2013, URL: http://sci.esa.int/solar-orbiter/50761-solar-orbiter-spacecraft/
27) Simon Barraclough, Oliver Merrill, Claudio Damasio, “Solar Orbiter Thermal Design Drivers and Verification Approach,” 41st International Conference on Environmental Systems
17 - 21 July 2011, Portland, Oregon, paper: AIAA 2011-5192, URL: http://enu.kz/repository/2011/AIAA-2011-5192.pdf
28) “Prehistoric cave pigment to shield ESA's Solar Probe,” ESA, February 12, 2014, URL: http://www.esa.int/Our_Activities/Space_Engineering/Prehistoric_cave_pigment_to_shield_ESA_s_solar_probe
29) L. Simone, N. Salerno, D. Gelfusa, V. Nanni, O. Cocciolillo, M. G. Campagna, “The X-band Deep Space Transponder for ExoMars and Solar Orbiter Missions,” Proceedings of TTC 2013, 6th International Workshop on Tracking Telemetry and Command Systems for Space Applications, Darmstadt, Germany, Sept. 10-13, 2013
30) ESA Bulletin No 161, May 11, 2015, p. 71, URL: http://esamultimedia.esa.int/multimedia/publications/ESA-Bulletin-161/offline/download.pdf
31) A. Lyngvi, N. Rando, L. Gerlach, A. Peacock, “The Solar Orbiter Thermal Design,” Proceedings of the 56th International Astronautical Congress, Oct. 17-21, 2005, Fukuoka, Japan , paper: IAC-05-C2.6.02, URL: http://sci.esa.int/science-e/www/object/doc.cfm?fobjectid=38256
32) ”Solar Orbiter liftoff,” ESA Science & Exploration, 10 February 2020, URL: http://www.esa.int/ESA_Multimedia/Videos/2020/02/Solar_Orbiter_liftoff
33) ”Orbiting the Sun together,” ESA / Science & Exploration / Space Science, 23 December 2019, URL: http://www.esa.int/Science_Exploration/Space_Science/Orbiting_the_Sun_together
34) ”Solar Orbiter, launch media kit,” ESA, 27 January 2019, URL: https://esamultimedia.esa.int/docs/science/solar_orbiter_media_kit.pdf
35) ”Solar Orbiter's journey around the Sun,” ESA, 17 October 2019, URL: http://www.esa.int/spaceinvideos/Videos/2019/10/Solar_Orbiter_s_journey_around_the_Sun
36) Daniel Müller, R. G. Marsden, O. C. St. Cyr, H. R. Gilbert, “Solar Orbiter - Exploring the Sun-Heliosphere Connection,” Online publication: Aug. 28,2012, Solar Physics, Vol. 285, Issue 1-2, pp. 25-70, July 2013, URL: http://www.solarorbiter.org/documents/presentations/overview/Solar_Orbiter_SPIE_Aug2013_dmueller.pdf
37) ”The Sun’s composition,” ESA Science & Exploration, 18 May 2022, URL: https://www.esa.int/var/esa/storage/images/esa_multimedia/images/2022/05/the_sun_s_composition/24071906-1-eng-GB/The_Sun_s_composition_pillars.png
38) ”Solar flare 2 March,” ESA Science & Exploration, 18 May 2022, URL: https://www.esa.int/ESA_Multimedia/Images/2022/05/Solar_flare_2_March2
39) ”The Sun as you’ve never seen it before,” ESA Science & Exploration, 18 May 2022, URL: https://www.esa.int/Science_Exploration/Space_Science/Solar_Orbiter/The_Sun_as_you_ve_never_seen_it_before
40) ”Tracking sunspots up close,” ESA Enabling & Support, 24 March 2022, URL: https://www.esa.int/ESA_Multimedia/Images/2022/03/Tracking_sunspots_up_close
41) ”Zooming into the Sun with Solar Orbiter,” ESA Science & Exploration, 24 March 2022, URL: https://www.esa.int/Science_Exploration/Space_Science/Solar_Orbiter/Zooming_into_the_Sun_with_Solar_Orbiter
42) ”Solar Orbiter crosses the Earth-Sun line as it heads for the Sun,” ESA Science & Exploration, 7 March 2022, URL: https://www.esa.int/Science_Exploration/Space_Science/Solar_Orbiter/Solar_Orbiter_crosses_the_Earth-Sun_line_as_it_heads_for_the_Sun
43) ”Giant solar eruption seen by Solar Orbiter,” ESA Science & Exploration, 18 February 2022, URL: https://www.esa.int/Science_Exploration/Space_Science/Solar_Orbiter/Giant_solar_eruption_seen_by_Solar_Orbiter
44) ”Solar Orbiter catches a second comet by the tail,” ESA Science & Exploration, 25 January 2022, URL: https://www.esa.int/Science_Exploration/Space_Science/Solar_Orbiter/Solar_Orbiter_catches_a_second_comet_by_the_tail
45) ”A Christmas comet for Solar Orbiter,” ESA Science & Exploration, 21 December 2021, URL: https://www.esa.int/Science_Exploration/Space_Science/Solar_Orbiter/A_Christmas_comet_for_Solar_Orbiter
46) ”Solar Orbiter publishes a wealth of science results from its cruise phase,” ESA Science & Exploration, 14 December 2021, URL: https://www.esa.int/Science_Exploration/Space_Science/Solar_Orbiter/Solar_Orbiter_publishes_a_wealth_of_science_results_from_its_cruise_phase
47) ”Solar Orbiter returns to Earth before starting its main science mission,” ESA Science & Exploration, 18 November, 2021, URL: https://www.esa.int/Science_Exploration/Space_Science/Solar_Orbiter/Solar_Orbiter_returns_to_Earth_before_starting_its_main_science_mission
48) ”Gravity assists: nature balances her books,” ESA Enabling & Support, 9 August 2021, URL: https://www.esa.int/Enabling_Support/Operations/Gravity_assists_nature_balances_her_books
49) ”ESA gets ready for double Venus flyby,” ESA Science & Exploration, 2 August 2021, URL: https://www.esa.int/Science_Exploration/Space_Science/ESA_gets_ready_for_double_Venus_flyby
50) ”Solar Orbiter’s multi-instrument view of a coronal mass ejection,” ESA Science & Exploration, 17 May 2021, URL: https://www.esa.int/Science_Exploration/Space_Science/Solar_Orbiter/Solar_Orbiter_images_first_coronal_mass_ejections
51) ”‘Campfires’ offer clue to solar heating mystery,” ESA Science & Exploration, 27 April 2021, URL: https://www.esa.int/Science_Exploration/Space_Science/Solar_Orbiter/Campfires_offer_clue_to_solar_heating_mystery
52) Y. Chen, D. Przybylski, H. Peter, H. Tian, F. Auchère, D. Berghmans, ”Transient small-scale brightenings in the quiet solar corona: A model for campfires observed with Solar Orbiter,” Astronomy & Astrophysics, Published: 26 April 2021, https://doi.org/10.1051/0004-6361/202140638, URL: https://www.aanda.org/articles/aa/pdf/forth/aa40638-21.pdf
53) D. Berghmans, F. Auchère, D. M. Long, E. Soubrié, M. Mierla, A. N. Zhukov, U. Schühle, P. Antolin, L. Harra, et al., ”Extreme-UV quiet Sun brightenings observed by the Solar Orbiter/EUI,” Astronomy & Astrophysics, Published: 22 April 2021, https://doi.org/10.1051/0004-6361/202140380, URL: https://www.aanda.org/articles/aa/pdf/forth/aa40380-21.pdf
54) ”ESA’s Solar Orbiter ducks behind the Sun,” ESA / Enabling & Support / Operations, 9 February 2021, URL: https://www.esa.int/Enabling_Support/Operations/ESA_s_Solar_Orbiter_ducks_behind_the_Sun
55) ”Solar Orbiter snaps Venus, Earth and Mars,” ESA Science & Exploration, 26 January 2021: URL: https://www.esa.int/ESA_Multimedia/Images/2021/01/Solar_Orbiter_snaps_Venus_Earth_and_Mars
56) ”Solar Orbiter prepares for festive Venus flyby,” ESA Science & Exploration, 17 December 2020, URL: https://www.esa.int/Science_Exploration/Space_Science/Solar_Orbiter/Solar_Orbiter_prepares_for_festive_Venus_flyby
57) ”Solar Orbiter: turning pictures into physics,” ESA Science & Exploration, 10 December 2020, URL: https://www.esa.int/Science_Exploration/Space_Science/Solar_Orbiter/Solar_Orbiter_turning_pictures_into_physics
58) ”Solar Orbiter releases first data to the public,” ESA Science & Exploration, 30 September 2020, URL: https://www.esa.int/Science_Exploration/Space_Science/Solar_Orbiter/Solar_Orbiter_releases_first_data_to_the_public
59) ”Closer than ever: Solar Orbiter’s first views of the Sun,” ESA Science & Exploration, 16 July 2020, URL: https://www.esa.int/ESA_Multimedia/Videos/2020/07/Closer_than_ever_Solar_Orbiter_s_first_views_of_the_Sun
60) ”Solar Orbiter’s first views of the Sun,” ESA Science & Exploration, 16 July 2020, URL: https://www.esa.int/Science_Exploration/Space_Science/Solar_Orbiter/Solar_Orbiter_s_first_views_of_the_Sun_image_gallery
61) ”Solar Orbiter sees ‘campfires’ on the Sun,” ESA Science & Exploration, 16 July 2020, URL: https://www.esa.int/ESA_Multimedia/Videos/2020/07/Solar_Orbiter_sees_campfires_on_the_Sun
62) ”Solar Orbiter ready for science despite COVID-19 setbacks,” ESA Science & Exploration, 29 June 2020, URL: http://www.esa.int/Science_Exploration/Space_Science/Solar_Orbiter/Solar_Orbiter_ready_for_science_despite_COVID-19_setbacks
63) ”Solar Orbiter makes first close approach to the Sun,” ESA Science & Exploration, 15 June 2020, URL: http://www.esa.int/Science_Exploration/Space_Science/Solar_Orbiter/Solar_Orbiter_makes_first_close_approach_to_the_Sun
64) ”Solar Orbiter to pass through the tails of Comet ATLAS,” ESA / Science & Exploration / Space Science / Solar Orbiter, 29 May 2020, URL: http://www.esa.int/Science_Exploration/Space_Science/Solar_Orbiter/Solar_Orbiter_to_pass_through_the_tails_of_Comet_ATLAS
65) ”First Solar Orbiter instrument sends measurements,” ESA Science & Exploration, 17 February 2020, URL: http://www.esa.int/Science_Exploration/Space_Science/Solar_Orbiter/First_Solar_Orbiter_instrument_sends_measurements
66) ”Solar Orbiter braves challenging early days,” ESA / Enabling & Support / Operations, 14 February 2020, URL: http://www.esa.int/Enabling_Support/Operations/Solar_Orbiter_braves_challenging_early_days
67) ”Here comes the Sun,” ESA Science & Exploration, 11 February 2020, URL: http://www.esa.int/ESA_Multimedia/Images/2020/02/Here_comes_the_Sun
68) Salma Fahmy, Giorgio Bagnasco, Anne Pacros, Kristin Wirth, “Solar Orbiter Payload Suite: A Hotbed of Innovation,” Proceedings of the 64th International Astronautical Congress (IAC 2013), Beijing, China, Sept. 23-27, 2013, paper: IAC-13-A3.5.2
69) Payload accommodation onboard Solar Orbiter - Annotated,” ESA, July 30, 2013, URL: http://sci.esa.int/solar-orbiter/50760-payload-accommodation-onboard-solar-orbiter-annotated/
70) A. Alvarez-Herrero, P. García Parejo, H. Laguna, J. Villanueva, J. Barandiarán, L. Bastide, M- Reina, M. Royo, “The polarization modulators based on liquid crystal variable retarders for the PHI and METIS instruments for the Solar Orbiter mission,” Proceedings of the ICSO (International Conference on Space Optics), Tenerife, Canary Islands, Spain, Oct. 7-10, 2014, URL: http://congrexprojects.com/Custom/ICSO/2014/Papers/3.%20Thursday%209%20October/Session%2010B%20Adaptive%20Active%20Optics/4.66598_Alvarez-Herrero.pdf
71) O. Grimm, M. Bednarzik, G. Birrer, N. Arnold, V. Commichau, G. Hurford, S. Krucker, O. Limousin, A. Meuris, “Performance and qualification of CdTe pixel detectors for the Spectrometer/Telescope for Imaging X-rays,” Journal of Instrumentation, Volume 10, February 9, 2015
72) Charles Koeck, Ralph Cordey, Steve Kemble, Ulrich Johann, “From Mercury to Jupiter: overview on solar system exploration missions at Astrium,” Proceedings of the 63rd IAC (International Astronautical Congress), Naples, Italy, Oct. 1-5, 2012, paper: IAC-12-A3.5.9
73) Joachim Woch, “Polarimetric and Helioseismic Imager (PHI),” MPS, Oct. 8, 2008, URL: http://www.mps.mpg.de/en/projekte/solar-orbiter/phi/
74) Achim Gandorfer, Sami K Solanki, Joachim Woch, Valentin Martinez Pillet, Alberto Alvarez Herrero, Thierry Appourchaux, “The Solar Orbiter Mission and its Polarimetric and
Helioseismic Imager (SO/PHI),” Journal of Physics: Conference Series 271 (2011) 012086, doi:10.1088/1742-6596/271/1/012086, URL: http://www.mps.mpg.de/dokumente/publikationen/solanki/c227.pdf
75) “Solar Orbiter Payload Assessment Document,” SCI-A/2004/175/AO, Issue 4, Rev. 1, Aug. 23, 2005, ESA/ESTEC; URL: http://sci.esa.int/science-e/www/object/doc.cfm?fobjectid=37356
76) M. Silva-López, J. A. Bonet-Navarro, A. Nuñez, A. Álvarez-Herrero, “Evaluation of the refocusing system of the polarimetric helioseismic Imager/Full Disk Telescopeof the Solar Orbiter mission,” Proceedings of the ICSO (International Conference on Space Optics), Tenerife, Canary Islands, Spain, Oct. 7-10, 2014, URL: http://congrexprojects.com/Custom/ICSO/2014/Papers/5.%20Posters/Poster%20Session%202/2.35.56569_Silva.pdf
77) J. Barandiaran, P. Zuluaga, A. B. Fernandez, I. Vera, D. Garranzo A. Nuñez, L. Bastide, M. T. Royo, A. Alvarez, “Solar Orbiter /PHI Full Disk Telescope entrance window mechanical mount,” Proceedings of the ICSO (International Conference on Space Optics), Tenerife, Canary Islands, Spain, Oct. 7-10, 2014, URL: http://congrexprojects.com/Custom/ICSO/2014/Papers/1.%20Tuesday%207%20October/Session%204B%20Gratings%20-%20Other%20Components/6.66273_Barandiaran.pdf
78) Carlos Dominguez-Tagle, Thierry Appourchaux, Jean-Jacques Fourmond, Anne Philippon, Jean-Christophe Le Clec’h, Mehdi Bouzit, Ilyass Azzoug, “Filtergraph Calibration for the Polarimetric and Helioseismic Imager,” Proceedings of the 29th ISTS (International Symposium on Space Technology and Science), Nagoya-Aichi, Japan, June 2-8, 2013, paper: 2013-k-46
79) U. Schühle, “SPICE - Spectral Imaging of the Coronal Environment,” MPS, March 24, 2010, URL: http://www.mps.mpg.de/en/projekte/solar-orbiter/spice/#team
80) P. Rochus, J.-P. Halain, E. Renotte, D. Berghmans, A. Zhukov, J-F. Hochedez, T. Appourchaux, F. Auchère, L. K. Harra, U. Schühle, R.Mercier, “The Extreme Ultraviolet Imager (EUI) onboard the Solar Orbiter mission,” Proceedings of the 60th IAC (International Astronautical Congress), Daejeon, Korea, Oct. 12-16, 2009, IAC-09.A3.4.10
81) Jean-Philippe Halaina, Pierre Rochus, Thierry Appourchaux, David Berghmans, Louise Harra, Udo Schühle, Frédéric Auchère, Andrei Zhukov, Etienne Renotte, Jean-Marc Defise, Laurence Rossi, Karl Fleury-Frenette, Lionel Jacques, Jean-François Hochedez, Ali Ben Moussa, “The technical challenges of the Solar-Orbiter EUI instrument,” Proceedings of SPIE, 'Space Telescopes and Instrumentation 2010: Ultraviolet to Gamma Ray,' Volume 7732, July 29, 2010, doi:10.1117/12.857976, URL: http://orbi.ulg.ac.be/bitstream/2268/91656/1/SPIE7732-26.pdf
82) J.-P. Halain, P. Rochus, E. Renotte, F. Auchère, D. Berghmans, L. Harra, U. Schühle, W. Schmutz, A. Zhukov, R. Aznar Cuadrado, F. Delmotte, C. Dumesnil, M. Gyo, T. Kennedy, R. Mercier, F. Verbeeck, M. Thome, K. Heerlein, A. Hermans, L. Jacques, A. Mazzoli, S. Meining, L. Rossi, J. Tandy, P. Smith, B. Winter, “The extreme UV imager of solar orbiter: from detailed design to flight model,” Proceedings of SPIE, 'Space Telescopes and Instrumentation 2014: Ultraviolet to Gamma Ray,' Volume 9144, Montréal, Quebec, Canada, June 22, 2014, doi:10.1117/12.2055207
83) J.-P. Halain, A. Mazzoli, P. Rochus, E. Renotte, Y. Stockman, D. Berghmans, A. BenMoussa, “EUV high resolution imager on-board Solar Orbiter: optical design and detector performances,” Proceedings of the ICSO (International Conference on Space Optics), Ajaccio, Corsica, France, Oct. 9-12, 2012, paper: ICSO-172, URL: http://congrex.nl/icso/2012/papers/FP_ICSO-172.pdf
84) E. Antonucci, V. Andretta, S. Cesare, A. Ciaravella, G. Doschek, S. Fineschi, S. Giordano, P. Lamy, D. Moses, G. Naletto, J. Newmark, L. Poletto, M. Romoli, S. Solanki, D. Spadaro, L. Teriaca, L. Zangrilli, “METIS, the Multi-Element Telescope for Imaging and Spectroscopy: an instrument proposed for the Solar Orbiter Mission,” Proceedings of the 7th ICSO (International Conference on Space Optics) 2008, Toulouse, France, Oct. 14-17, 2008, URL: http://www.mps.mpg.de/dokumente/publikationen/solanki/c208.pdf
85) G. Naletto, E. Antonucci, V. Andretta, E. Battistelli, S. Cesare, V. Da Deppo, F. d’Angelo, S. Fineschi, M. Focardi, P. Lamy, F. Landini, D. Moses, G. Nicolini, P. Nicolosi, M. Pancrazzi, M-G. Pelizzo, L. Poletto, M. Romoli, S. Solanki, D. Spadaro, L. Teriaca, M. Uslenghi, L. Zangrilli, “METIS, the Multi Element Telescope for Imaging and Spectroscopy for the Solar Orbiter Mission,” ICSO 2010 (International Conference on Space Optics), Rhodes Island, Greece, Oct. 4-8, 2010, URL: http://congrex.nl/icso/Papers/Session%204b/FCXNL-10A02-1984829-1-NALETTO_ICSO_PAPER.pdf
86) F. Landini, S. Vives, M. Romoli, C. Guillon, M. Pancrazzi, C. Escolle, M. Focardi, S. Fineschi, E. Antonucci, G. Nicolini, G. Naletto, P. Nicolosi, D. Spadaro, “The optimization of the inverted occulter of the Solar Orbiter/METIS coronagraph/spectrometer,” Proceedings of the ICSO (International Conference on Space Optics), Ajaccio, Corsica, France, Oct. 9-12, 2012 , paper: ICSO-089, URL: http://congrex.nl/icso/2012/papers/FP_ICSO-089.pdf
87) M. Romoli, F. Landini, E. Antonucci, V. Andretta, A. Berlicki, S. Fineschi, J. D Moses, G. Naletto, P. Nicolosi, G. Nicolini, D. Spadaro, L. Teriaca, C. Baccani, M. Focardi, M. Pancrazzi, S. Pucci, L. Abbo, A. Bemporad, G. Capobianco, G. Massone, D. Telloni, E. Magli, V. Da Deppo, F. Frassetto, M. G. Pelizzo, L. Poletto, M. Uslenghi, S. Vives, M. Malvezzi, “METIS: The visible and UV coronagraph for Solar Orbiter,” Proceedings of the ICSO (International Conference on Space Optics), Tenerife, Canary Islands, Spain, Oct. 7-10, 2014, URL: http://congrexprojects.com/Custom/ICSO/2014/Papers/2.%20Wednesday%208%20October/Session%206C%20Sci%20and%20Focal%20Plane%20Instruments/3.67021_Romoli.pdf
88) E. Verroi, V. Da Deppo, G. Naletto, S. Fineschi, E. Antonucci, “METIS- ESA Solar Orbiter mission internal straylight analysis,” Proceedings of the ICSO (International Conference on Space Optics), Tenerife, Canary Islands, Spain, Oct. 7-10, 2014, URL: http://congrexprojects.com/Custom/ICSO/2014/Papers/2.%20Wednesday%208%20October/Session%206C%20Sci%20and%20Focal%20Plane%20Instruments/4.67248_Verroi.pdf
89) F. Frassetto, L. Poletto, S. Fineschi, C. De Santi, M. Meneghini, G. Meneghesso, E. Antonucci, G. Naletto, M. Romoli, D. Spadaro, G. Nicolini, “Internal checkup illumination sources for METIS coronagraph on Solar Orbiter,” Proceedings of the ICSO (International Conference on Space Optics), Tenerife, Canary Islands, Spain, Oct. 7-10, 2014, URL: http://congrexprojects.com/Custom/ICSO/2014/Papers/5.%20Posters/Poster%20Session%202/2.14.68312_Frassetto.pdf
90) M. Romoli, F. Landini, D. Moses, S. Fineschi, M. Pancrazzi, M. Focardi, E. Antonucci, G. Nicolini, G. Naletto, P. Nicolosi, D. Spadaro, “Evaluation of the stray light from the diffraction of METIS coronagraph external occulter,” Proceedings of the ICSO (International Conference on Space Optics), Ajaccio, Corsica, France, Oct. 9-12, 2012, paper: ICSO-093, URL: http://congrex.nl/icso/2012/papers/FP_ICSO-093.pdf
91) A. Benz, S. Krucker, G. Hurford, A. Veronig, and the STIX Team, “X-ray imaging of the flaring corona with STIX ( Spectrometer/Telescope for Imaging X-rays),” URL: http://solarorbiter3.oato.inaf.it/Presentazioni/so3_2805_benz_talk.pdf
92) Oliver Grimm for the STIX collaboration, “The Spectrometer Telescope for Imaging X-Rays on-board the ESA Solar Orbiter satellite,” 13th Vienna Conference on Instrumentation, Feb. 12, 2013, Vienna, Austria, URL: http://indico.cern.ch/getFile.py/access?contribId=79&sessionId=7&resId=1&materialId=slides&confId=186337
93) A. O. Benz, S. Krucker, G. J. Hurford, N. G. Arnold, P. Orleanski, H.-P. Gröbelbauer, S. Klober, L. Iseli ,H. J. Wiehl, A. Csillaghy, L. Etesi, N. Hochmuth, M. Battaglia, M. Bednarzik, R. Resanovic, O. Grimm, G. Viertel, V. Commichau, A. Meuris, O. Limousin, S. Brun, N. Vilmer, K. R. Skup, R. Graczyk, M. Stolarski, M. Michalska, W. Nowosielski ,A. Cichocki, M. Mosdorf, K. Seweryn, A. Przepiórka, J. Sylwester, M. Kowalinski, T. Mrozek, P. Podgorski, G. Mann, H. Aurass, E. Popow, H. Önel, F. Dionies, S. Bauer, J. Rendtel, A. Warmuth, M. Woche, D. Plüschke, W. Bittner, J. Paschke, D. Wolker, H. F. Van Beek, F. Farnik, J. Kasparova, A. M. Veronig, I. W. Kienreich, P. T. Gallagher, D. S. Bloomfield, M. Piana, A. M. Massone, B. R. Dennis, R. A. Schwarz, R. P. Lin, “The spectrometer telescope for imaging x-rays on board the Solar Orbiter mission,” Proceedings of SPIE, 'Space Telescopes and Instrumentation 2012: Ultraviolet to Gamma Ray, 84433L, Amsterdam, The Netherlands, July 1, 2012, Vol. 8443, (September 7, 2012); doi:10.1117/12.927793, URL of Poster: http://www.esep.pro/sites/esep/IMG/pdf/poster_spie12_stix_8443-131_v3.pdf
94) Manolis K. Georgoulis, Athanassios C. Katsiyannis, Thomas G. Chondros, Athanassios Skodras, Costantinos Gontikakis, “Greek Participation to Solar Orbiter Development,” URL: [web source no longer available]
95) AIP (Astrophysikalisches Institut Potsdam), URL: http://www.aip.de/groups/osra/english/en_solo.html
96) “NRL's SoloHI Instrument Selected for Flight on Solar Orbiter Mission,” NRL, Jan. 23.2012, URL: http://www.nrl.navy.mil/media/news-releases/2012/nrls-solohi-instrument-selected-for-flight-on-solar-orbiter-mission
97) Angelos Vourlidas and the SoLOHI team, “Heliospheric Imaging: Linking the Corona with the Solar Wind,” URL: http://solarorbiter3.oato.inaf.it/Presentazioni/so3_2805_vourlidas_talk.pdf
98) Russell A. Howard, Angelos Vourlidas, Clarence M. Korendyke, Simon P. Plunkett, Michael T. Carter, Dennis Wang, Nathan Rich, Donald R. McMullin, Sean Lynch, Adam Thurn, Greg Clifford, Dennis G. Socker, Arnaud F. Thernisien, Damien Chua, Mark G. Linton, David Keller, James R. Janesick, John Tower, Mark Grygon, Robert Hagood, William Bast, Paulett C. Liewer, Eric M. DeJong, Marco M. C. Velli, Zoran Mikic, Volker Bothmer, Pierre Rochus, Jean-Philippe Halain, Philippe L. Lamy, “ The solar and heliospheric imager (SolOHI) instrument for the solar orbiter mission,” Proceedings of SPIE, 'Solar Physics and Space Weather Instrumentation V,' 88620H, San Diego, CA, USA, August 25, 2013, (September 26, 2013) ; doi:10.1117/12.2027657
99) “SRI International and TowerJazz Deliver First SoloHI CMOS Imagers to the Naval Research Laboratory for Solar Orbiter Mission,” SRI International, Oct. 13, 2013, URL: http://www.sri.com/newsroom/press-releases/sri-towerjazz-deliver-first-solohi-cmos-imagers-nrl
100) C. J. Owen, P. Louarn, S. Livi, “SWA: The Solar Wind L’Aquila Summer School 2014,” URL: http://www.cifs-isss.org/presentazioni/2014-september/Owen.pdf
101) “The in-situ instruments,” ESA, URL: http://sci.esa.int/solar-orbiter/51217-instruments/
102) “PAS - Proton-Alpha Sensor,” URL: http://www.ucl.ac.uk/mssl/space-plasma-physics/missions/solar-orbiter/swa-sensors/swa-pas
103) http://www3.imperial.ac.uk/spat/research/missions/space_missions/solar_orbiter
104) “Solar Orbiter's Energetic Particle Detector (EPD),” CAU/IEAP, URL: http://www.ieap.uni-kiel.de/et/solar-orbiter/
105) Ronald Castillo, Javier Almena, Alberto Carrasco, Aaron Montalvo, Oscar Gutiérrez, Manuel Prieto, Sebastián Sánchez, “Implementation and use of SpaceWire in the EPD instrument for Solar Orbiter,” Proceedings of the 5th International SpaceWire Conference 2013, Gothenburg, Sweden, June 10 - 14, 2013, pp: 222-225, URL: http://2013.spacewire-conference.org/downloads/SpW2013ProceedingsFinal.pdf
106) “Solar Orbiter's Energetic Particle Detector (EPD) HET,” URL: http://www.ieap.uni-kiel.de/et/solar-orbiter/HET.php
107) “Solar Orbiter's Energetic Particle Detector (EPD) - LET,” URL: http://www.ieap.uni-kiel.de/et/solar-orbiter/LET.php
108) Micheala Sosby, Rob Garner, ”New Views of Sun: 2 Missions Will Go Closer to Our Star Than Ever Before,” NASA, 16 May 2018, URL: https://www.nasa.gov/feature/goddard/2018/new-views-of-sun-2-missions-will-go-closer-to-our-star-than-ever-before
The information compiled and edited in this article was provided by Herbert J. Kramer from his documentation of: ”Observation of the Earth and Its Environment: Survey of Missions and Sensors” (Springer Verlag) as well as many other sources after the publication of the 4th edition in 2002.
Spacecraft Launch Mission Status Sensor Complement References Back to top