SAC-D (Satélite de Aplicaciones Científicas-D)/Aquarius Mission
EO
Multiple direction/polarisation radiometers
Atmosphere
Ocean
Quick facts
Overview
Mission type | EO |
Agency | NASA, CONAE |
Mission status | Mission complete |
Launch date | 10 Jun 2011 |
End of life date | 08 Jun 2015 |
Measurement domain | Atmosphere, Ocean, Land, Gravity and Magnetic Fields, Snow & Ice |
Measurement category | Liquid water and precipitation rate, Atmospheric Temperature Fields, Multi-purpose imagery (land), Surface temperature (land), Gravity, Magnetic and Geodynamic measurements, Surface temperature (ocean), Atmospheric Humidity Fields, Sea ice cover, edge and thickness, Ocean surface winds, Lightning Detection, Ocean Salinity |
Measurement detailed | Atmospheric pressure (over sea surface), Precipitation intensity at the surface (liquid or solid), Fire temperature, Atmospheric specific humidity (column/profile), Land surface temperature, Sea surface temperature, Sea-ice cover, Wind speed over sea surface (horizontal), Gravity field, Active Fire Detection, Total lightning density, Atmospheric pressure (over land surface), Water vapour imagery, Sea Surface salinity |
Instruments | MWR, ROSA, TDP, Aquarius L-Band Scatterometer, HSC (SAC-D/Aquarius), Aquarius L-Band radiometer, CARMEN-1 (ICARE), DCS (SAC-D), NIRST, Lagrange, CARMEN-1 (SODAD) |
Instrument type | Multiple direction/polarisation radiometers, Imaging multi-spectral radiometers (vis/IR), Space environment, Scatterometers, Precision orbit, Imaging multi-spectral radiometers (passive microwave), Data collection, Atmospheric temperature and humidity sounders |
CEOS EO Handbook | See SAC-D (Satélite de Aplicaciones Científicas-D)/Aquarius Mission summary |
SAC-D (Satélite de Aplicaciones Científicas-D)/Aquarius Mission
Overview Spacecraft Launch Mission Status Sensor Complement Ground Segment References
SAC-D/Aquarius is a cooperative international mission between CONAE (Comisión Nacional de Actividades Espaciales), Argentina, and NASA, USA. NASA uses the term Aquarius for the mission within its ESSP (Earth System Science Pathfinder) program (where Aquarius happens to be the prime instrument of the mission). At CONAE, which provides the spacecraft, the mission is referred to as SAC-D (Scientific Application Satellite-D) in reference to its previous missions. On March 3, 2004, the Memorandum of Understanding (MOU) for SAC-D/Aquarius construction was signed by Jorge Taiana, vice-chairman of the Board of the Argentine Space Agency CONAE, and the Administrator of NASA, Sean O'Keefe. The MOU was signed in Buenos Aires giving formal status to the decision to construct fully in Argentina the joint space mission SAC-D/Aquarius. 1) 2) 3) 4) 5) 6) 7) 8) 9) 10) 11) 12) 13)
In addition, instrument contributions are provided from ASI (Agenzia Spaziale Italiana) of Italy, CNES (Center National d'Etudes Spatiales) of France, and CSA (Canadian Space Agency) of Canada.
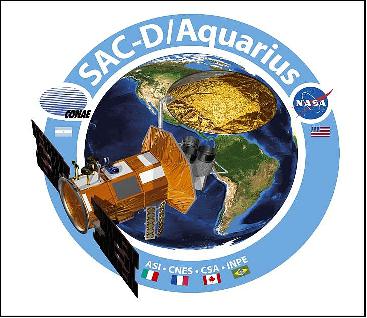
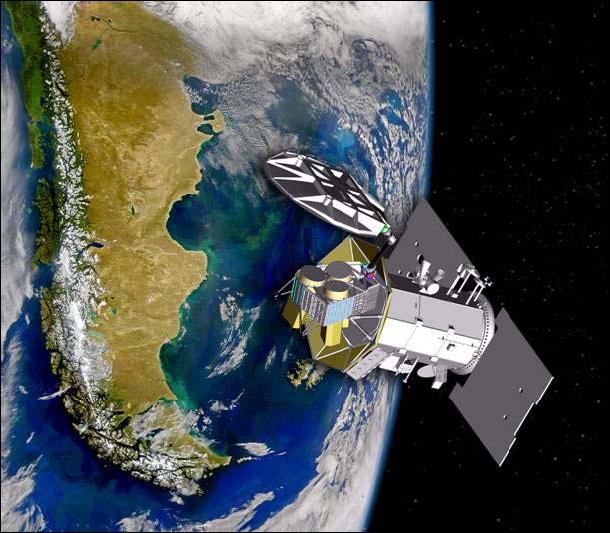
SAC-D/Aquarius is a multi-sensor mission covering ocean, land, atmosphere and space environments (Table 1). The main objective is to contribute to the understanding of the total Earth system and the consequences of the natural and man-made changes in the environment of the planet. The primary science goal is to study the processes that couple changes in the water cycle and ocean circulation, and influence present and future climate, by measuring sea surface salinity (SSS) variations globally for at least three years. Additional objectives include: 14) 15)
• Monitoring environmental changes, natural hazards and sea ice
- Measurement of SSS (Sea Surface Salinity)
- Measurement of Sea Surface Temperature (SST)
• Monitoring atmospheric parameters
- Measurement of the temperature and humidity profile of the troposphere and the stratosphere
- Measurement of rain rates, surface wind speeds, water vapor and cloud liquid water, over the oceans
- Measurement of sea ice concentration
• Studying effect of cosmic radiation on electronic devices and characteristics of space debris.
The prime instrument of the mission, Aquarius, provides maps of salt concentration on the ocean surface. The data is needed to study the heat capacity of the ocean's which in turn effects Earth's climate and the water cycle.
The Aquarius science goals are to observe and model the processes that relate salinity variations to climatic changes in the global cycling of water and to understand how these variations influence general ocean circulation. Accurate measurements of SSS (Sea Surface Salinity), along with sea surface temperature, will determine the sea surface density, which controls the formation of water masses and regulates the 3-dimensional ocean circulation. The goals of SAC-D/Aquarius are closely aligned to the goals of NASA's ESE (Earth Science Enterprise) program and to the National Space Program of Argentina.
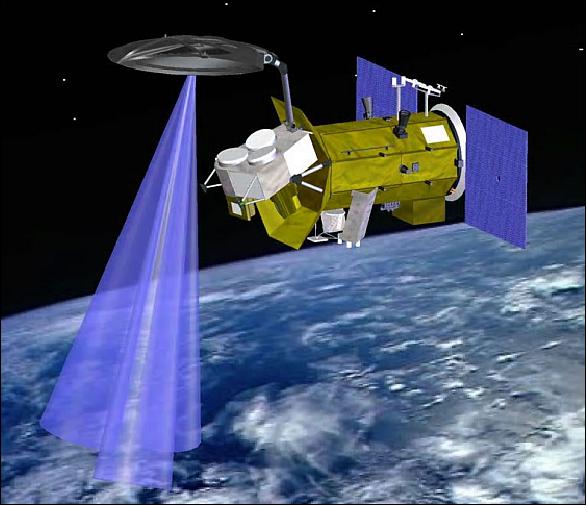
SAC-D/Aquarius is a Principal Investigator (PI) mission organized under the ESSP-3 initiative of NASA [PIs: Gary S. E. Lagerloef of ESR (Earth & Space Research), Seattle, WA; Raul F. Colomb of CONAE, Argentina; Note: since mid-2008 Sandra Torrusio is the PI successor of Raul F. Colomb who passed away in May 2008]. In the overall agreements, the spacecraft is being provided by CONAE based on the SAC-C platform design. CONAE provides in addition several payload instruments. The spacecraft mission operations are also part of CONAE, consisting of two elements: 16)
1) The ground station and mission operations center located in Cordoba, Argentina
2) The instrument data distribution facility located in Buenos Aires, Argentina (raw data archive).
NASA (GSFC, JPL) is providing the development of the primary payload (Aquarius) and its integration, the launch of the spacecraft, and the data processing system of Aquarius. There are also payload contributions (third party instruments) from Italy, France, and Canada.
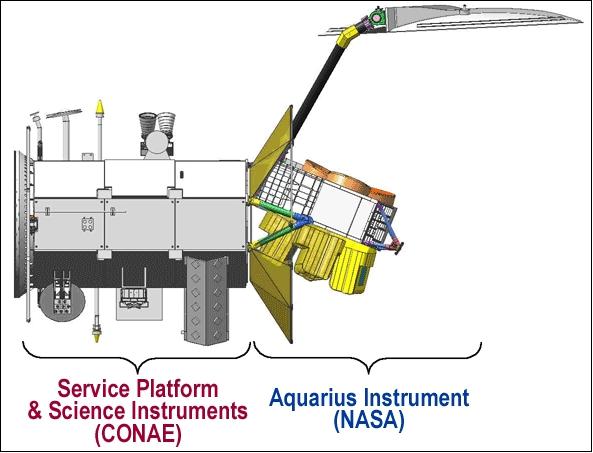
Instrument | Objectives | Specification | Resolution | Owner |
Aquarius | Understanding ocean circulation, global water cycle and climate interaction. Soil moisture measurements over Argentina | Integrated L-band radiometers (1..413 GHz) and scatterometer (1.26 GHz) | Three beams: | NASA |
MWR | Precipitation rate, wind speed, sea ice concentration, water vapor, clouds | 23.8 and 37 GHz, H+V polarization; Bandwidth: 0.5 and 1 GHz; Swath: 390 km | 40 km | CONAE |
NIRST | Hot spot events, SST (Sea Surface Temperature) measurements | 3.8, 10.7 and 11.7 µm bands; Swath of 180 km | 350 m | CONAE, |
HSC | Urban lights, electric storms, polar regions, snow cover | 0.45 - 0.9 µm | 200-300 m | CONAE |
DCS | Data Collection System | 401.55 MHz uplink | 2 contacts/day from 200 platforms | CONAE |
ROSA | Atmospheric properties | GPS occultation techniques | 300 km horizontal | ASI (Italy) |
CARMEN-1 | I: Effect of cosmic radiation in electronic devices, S: distribution of microparticles and space debris | I: three fully depleted Si and Si/Li detectors | I: 256 channels | CNES (France) |
TDP (Technology Demonstration Package) | Position, velocity and time determination, inertial angular velocity | GPS receiver, | 20 m, 1 m/s, 2 m/s | CONAE |
Spacecraft
The SAC-D spacecraft of CONAE is of SAC-C heritage using the SSSP (Small Satellite Standard Platform) bus built by INVAP (Investigaciones Aplicadas) at the Patagonian town of Bariloche for CONAE (INVAP is the prime contractor of the SAC-D project for CONAE).
The spacecraft is 3-axis stabilized using redundancy at the functional and unit level. The platform is Earth-oriented using maneuvering thrusters for orbit maintennance. Attitude sensing is provided by 2 star trackers, 2 magnetometers, 12 coarse sun sensors, and 2 GPS receivers; actuation is provided by 4 reaction wheels and 3 torque rods. Additional spacecraft characteristics are summarized in Table 2. 17) 18) 19)
The spacecraft has a launch mass of 1515 kg including 65 kg of fuel (launch configuration of 2.74 m x 4.85 m) and a design life of 3 years for Aquarius and 5 years for the SAC-D instruments. The spacecraft bus has a mass of 800 kg, the Aquarius assembly mass is 375kg, and the other SAC-D instruments have a mass of 275 kg (Ref. 22).
Parameter | Value | Parameter | Value |
Structure material | Aluminum, sandwich | Thermal control | Passive heaters |
Propulsion type | Monopropellant hydrazine | No of thrusters | 8 thrusters, 1 tank |
Attitude control method | three-axis stabilized | Attitude control ref. | Stars |
Attitude control capability | 0.04º (3σ) | Attitude knowledge | 0.002º (3σ) |
S/C TT&C data rate | 1 kbit/s | Onboard data storage | Mass memory, 768 Mbit |
Max storage recording | 64 kbit/s | Max playback rate | 5 Mbit/s |
Power array structure | fixed, 3 panels, 1 body | Solar array size | 5.2 m2, 3.25 m x 1.6 m |
Solar cell type | multi-junction GaAs | Solar cell efficiency | 27% |
Power generation | BOL=1.6 kW EOL=1.48 kW | Average power consumption | 699 W |
Battery type | NiCd | Battery storage capacity | 50 Ah |
Bus voltage | 28 V | S/C design life | 3 years (5 years goal) |
Launch
The SAC-D/Aquarius spacecraft was launched on June 10, 2011 on a Delta 2 rocket vehicle (7320-10C shroud) from VAFB, CA, USA. The launch provider was ULA (United Launch Alliance). This launch represented the final flight of the Delta II 7300 series. 20)
The launch was delayed from May 2010 because development of the spacecraft was taking longer than expected. 21)
Orbit: Sun-synchronous circular orbit, altitude = 657 km, inclination = 98º, the nodal crossing is at 18:00 hours on the ascending node (dawn/dusk orbit). This will enable complete Earth coverage every 7 days (exact repeat after 103 orbits).
RF communications: The spacecraft communications subsystem provides for both S-band and X-band upload and download. There are redundant S-band and X-band transceivers. S-band is used for TT&C communications.
Four of the SAC-D instruments (MWR, NIRST, DCS, and ROSA) needed to be interfaced with the PAD (Payload Data Distribution) electronics that accommodates the higher science data rates of these instruments. The PAD receives each instrument's data, formats the data streams, and inserts the data into a virtual channel of 4.0 Mbit/s that is passed through the mass memory unit and onto the X-band telecommunication system for downlink over scheduled ground stations.
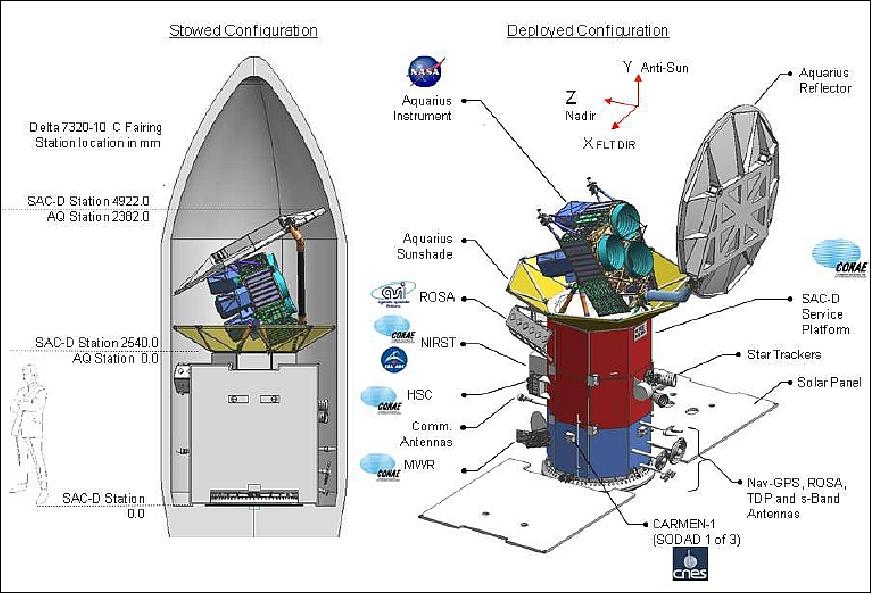
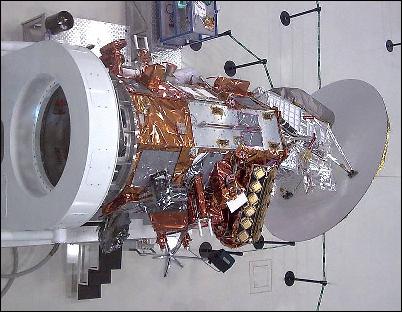
Mission Status
• The study of the salinity of the ocean surface ended on June 8, 2015, when an essential part of the power and attitude control system for the SAC-D spacecraft, which carries NASA's Aquarius instrument, stopped operating. The Aquarius instrument successfully achieved its science objectives and completed its primary three-year mission in November 2014. 23)
- Aquarius was a pathfinder mission to demonstrate that accurate, scientifically significant measurements of salinity could be made from space. It was also the first mission to combine use of passive (radiometer) and active (radar) measurements at L-band.
- "The Aquarius sensor collected three years and nine months of valuable data," said Aquarius principal investigator Gary Lagerloef of Earth & Space Research, Seattle. "It was truly a pioneering effort to determine how accurately we could measure ocean salinity from space and for the first time study large and small-scale interactions of the global water cycle."
- Preliminary indications are that an onboard hardware component called RTU (Remote Terminal Unit) shut down, which caused the loss of onboard power regulation and spacecraft attitude stabilization.
- Salinity information is critical to improving our understanding of two major components of Earth's climate system: the water cycle and ocean circulation. By measuring ocean salinity from space, Aquarius provided new insights into the massive natural exchange of freshwater between the ocean, atmosphere and sea ice, which - in turn - influences ocean circulation, weather and climate.
- Data from Aquarius revealed how extreme floods impact our seas and how low-salinity river plumes affect hurricane intensity. Aquarius data also were integral to SPURS (Salinity Processes in the Upper Ocean Regional Study), a year-long international field study of the oceanographic processes that sustain the maximum surface salinities in the central subtropical North Atlantic, and influence global ocean circulation.
• November 2014: The Aquarius/SAC-D satellite mission convened the 9th Science Meeting in Seattle, WA, USA, 11-14 November 2014. This was be the 3rd science meeting since the mission was launched 10 June 2011. The meeting was scheduled to coincide with the completion of NASA's Aquarius 3-year prime salinity measurement mission in November. The agenda emphasized the scientific achievements of these three years, validating the NASA mission science requirements, and to form future plans for Aquarius extended mission science (NASA Senior Review in 2015). The meeting focused on the Aquarius/SAC-D science team and the NASA Ocean Salinity Science Team (OSST) activities. 24)
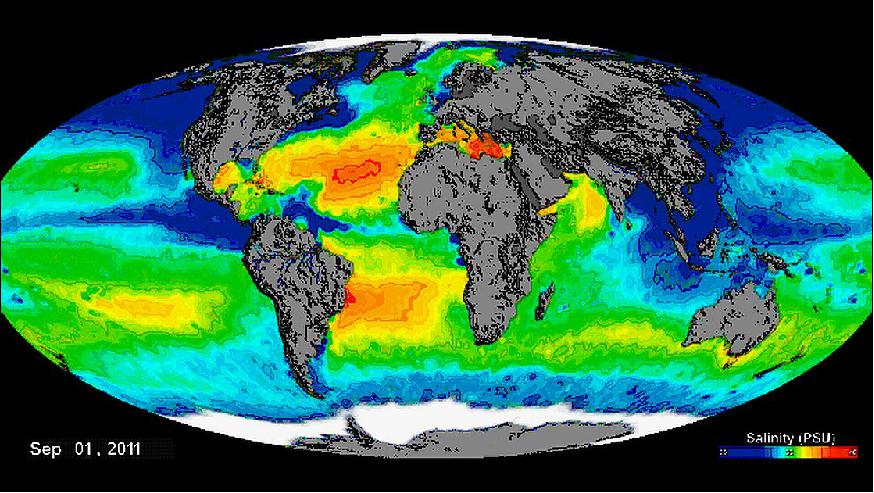
• 2014: The Aquarius/SAC-D satellite has been collecting SSS (Sea Surface Salinity) measurements since Aquarius instrument commissioning was completed on August 25, 2011, and has produced 35 months of data. The mission‘s overarching goal is to map sea surface salinity and its changes over space and time to study the links between ocean circulation, the global water cycle, and climate. Early science objectives include mapping the global mean annual SSS field and seasonal cycle with significantly better spatial resolution than is possible with sparsely sampled in situ observations. The primary science requirement is to map SSS with monthly average measurement error less than 0.2 PSU (practical salinity scale), and 150 km spatial resolution. 26)
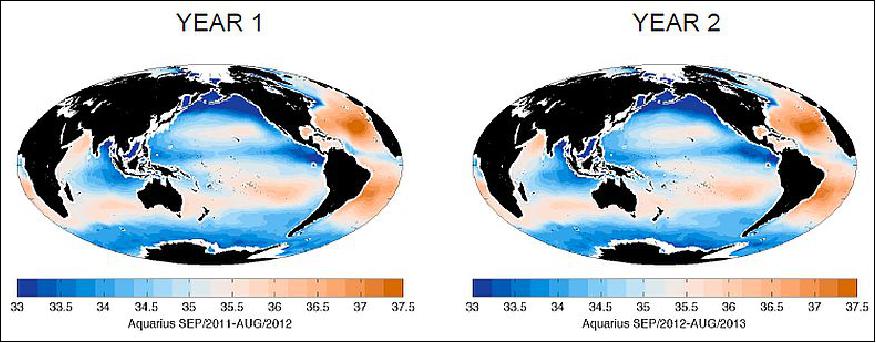
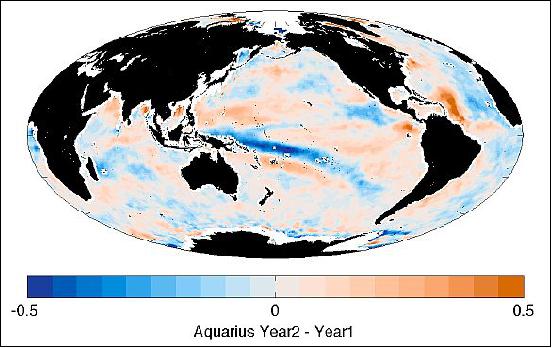
• July 2014: The Aquarius instrument on SAC-D was built to study the salt content of ocean surface waters. The new soil wetness measurements were not in the mission's primary science objectives, but a NASA-funded team led by USDA (U.S. Department of Agriculture) researchers has developed a method to retrieve soil moisture data from the instrument's microwave radiometer. 27)
Soils naturally radiate microwaves and the Aquarius sensor can detect the microwave signal from the top 5 cm of the land, a signal that subtly varies with changes in the wetness of the soil. Aquarius takes eight days to complete each worldwide survey of soil moisture, although with gaps in mountainous or vegetated terrain where the microwave signal becomes difficult to interpret.
Soil moisture, the water contained within soil particles, is an important player in Earth's water cycle. It is essential for plant life and influences weather and climate. Satellite readings of soil moisture will help scientists better understand the climate system and have potential for a wide range of applications, from advancing climate models, weather forecasts, drought monitoring and flood prediction to informing water management decisions and aiding in predictions of agricultural productivity.
USDA uses soil wetness information to improve crop forecasts. These forecasts not only help farmers and markets adjust their prices according to worldwide production, but also allow relief agencies to plan for food emergency responses.
Aquarius soil moisture data are coarser than those collected by the SMOS (Soil Moisture and Ocean Salinity) mission of ESA. Still, having multiple missions in orbit that simultaneously measure the wetness of the land in the same band of the microwave spectrum ensures a more continuous record.
NASA's SMAP (Soil Moisture Active/Passive) mission, due for launch in November 2014, will advance soil moisture studies with its greater spatial and temporal resolution. The new mission will combine microwave radiometer readings, which are accurate but coarse, with the measurements taken by its onboard radar, which are less precise but have higher spatial resolution than the radiometer data. This approach will provide a footprint of 9 km, and it will produce worldwide soil moisture maps every three days. Taken together, these features of SMAP will provide about 500 times the number of soil moisture measurements per day compared to SMOS or Aquarius.
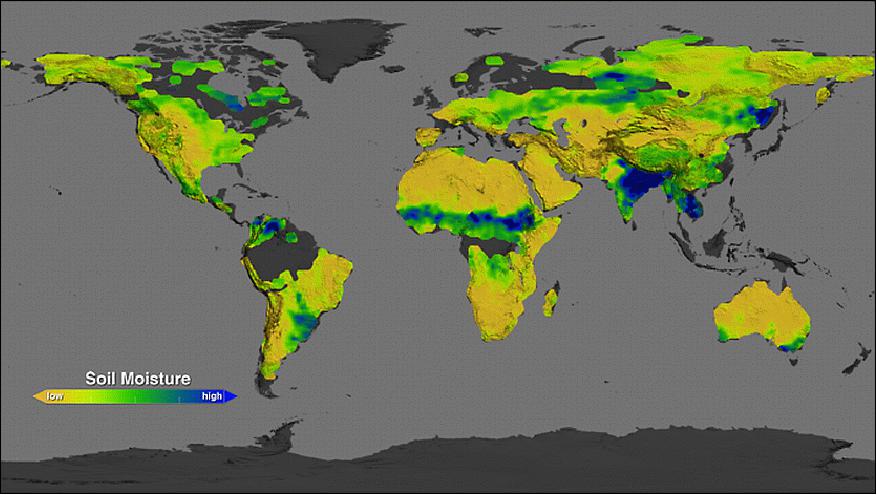
• In early 2014, the SAC-D/Aquarius mission is operating nominally, (2 ½ years on orbit). 28)
- Aquarius data are also now being used to monitor global soil moisture conditions. Soil moisture products, derived from Aquarius's L-band horizontally polarized brightness temperature observations, were first made available by NSIDC (National Snow and Ice Data Center) in December 2013.
For example, Figure 11 shows two Aquarius soil moisture maps centered on the Indian subcontinent: one from May 2012; the other from August 2012. The map from May is marked by dry soil conditions in India, while the map from August shows saturated conditions from monsoon rains.
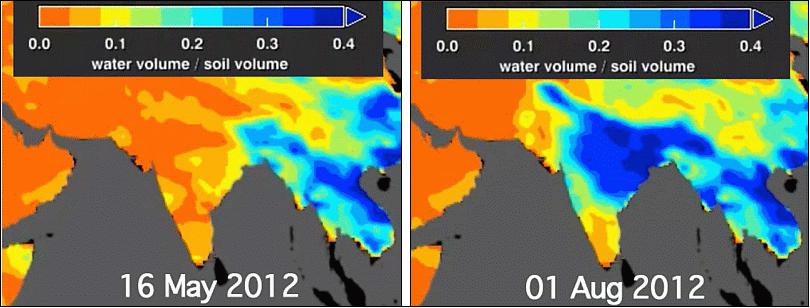
- Calibration and validation of the Aquarius instrument continues to be an active area of research at the current phase of the mission. For Aquarius investigators—and the microwave remote sensing community in general—one "byproduct" of Aquarius measurements is proving to be important: global maps of RFI (Radio Frequency Interference). Ironically, RFI at L-band is a problem that should not exist, at least for Aquarius, since its radiometers operate at a band centered at 1.41 GHz, which is "protected" by international agreements for use in radio astronomy. Employing this frequency permits Aquarius to detect the small brightness temperature signals naturally emitted from Earth's surface. However, even very weak out-of-band signals from man-made sources, such as communications and air traffic control radar, can overwhelm the natural signal that Aquarius is designed to measure.
Aquarius instrument designers and builders have taken special precautions to detect and mitigate RFI. Rapid sampling and a "glitch-detection" algorithm have worked well. The detected RFI is removed before processing but is used to make maps of the location and extent of RFI, which helps the research community to mitigate the problem. Among Aquarius' novel features is that it produces RFI maps not only for its radiometers, but also for the scatterometer (1.26 GHz). The problem is in some ways worse for the scatterometer because it operates in a spectral band shared with other radar instruments, in particular those used for air traffic control.
Figure 12 shows two examples of these maps, which clearly illustrate that the U.S. is relatively clean in terms of RFI that adversely impacts the radiometer; however, in the scatterometer band, the same area is overwhelmed with signals from air traffic control radar.
![Figure 12: These maps show the location of RFI over North America during August 2013 as measured by the Aquarius' radiometers (1.41 GHz) [top] and scatterometer (1.26 GHz) [bottom], image credit: NASA (Ref. 28)](/api/cms/documents/163813/1717893/SACD_Auto1B.jpeg)
• May 2013: The Aquarius sensor and the SAC-D observatory continue to perform well. During much of the second half of 2012 there were interruptions in the precise geodetic pointing, and some safe hold events. These occurred during the first and last quarters of the lunar cycle as the moonlight sometimes interfered with the star tracker. In November, 2012 the Flight Operations Team implemented ways to mitigate this problem and it has since been under control. A number of cold-sky calibration maneuvers have also been done in recent months. 29)
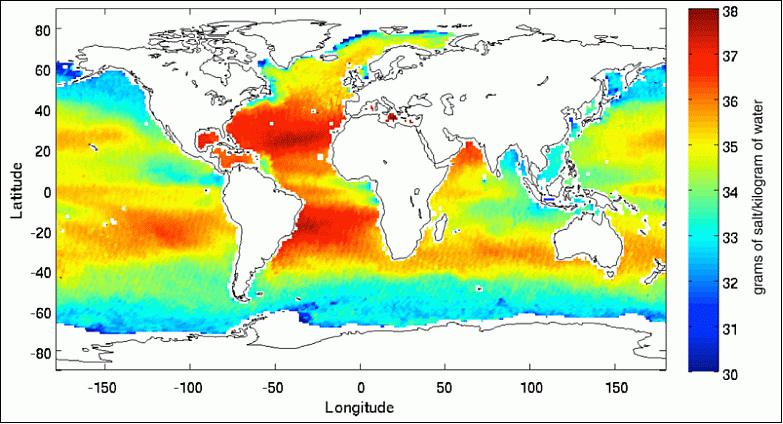
• In February 2013, the SAC-D spacecraft is operating nominally. The instruments Aquarius, MWR, HSC, Carmen 1, and DCS are fully operative. ROSA is still under specific analysis by ASI, it is expected to be operational soon. The Aquarius and MWR data are available to the user community. 30)
Note: A subset of ROSA products have been validated against ECMWF data and compared with COSMIC co-located events in order to verify the bending angles, temperature refractivity profiles performances. Moreover, total electron contents were analyzed and compared with COSMIC collocated events, showing a satisfactory agreement (Ref. 68).
• On June 10, 2012, the SAC-D/Aquarius observatory celebrated its first year on orbit. NASA's Aquarius instrument has been measuring changes in salinity, or salt concentration, in the surface layer of the oceans. 31) 32)
An overarching question in climate research is to understand how changes in the Earth's water cycle – meaning rainfall and evaporation, river discharges and so forth – ocean circulation, and climate link together. Most global precipitation and evaporation events take place over the ocean and are very difficult to measure. But rainfall freshens the oceans' surface waters, and Aquarius can detect these changes in saltiness.
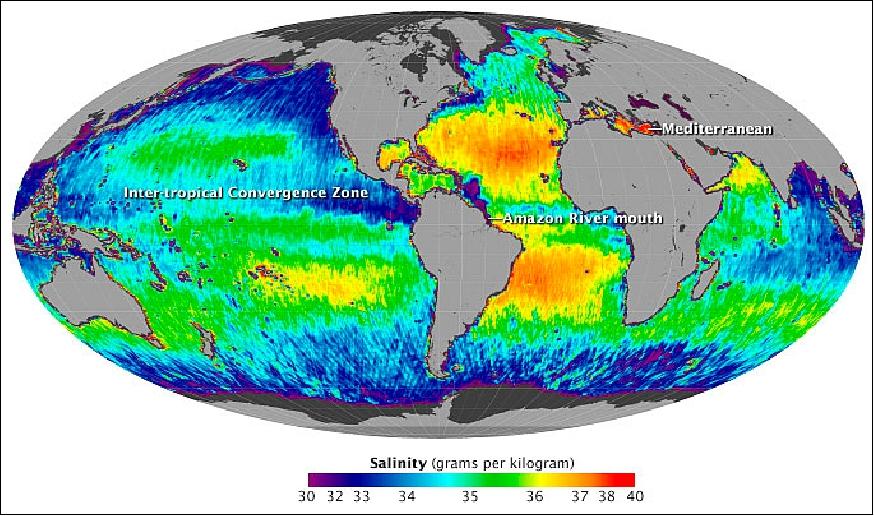
• In April/May 2012, the SAC-D spacecraft and the following instruments are operating nominally: 34)
- Aquarius of NASA
- MWR (Microwave Radiometer)
- HSC (High Sensitivity Camera)
- DCS (Data Collection System)
- ROSA (Radio Occultation Sounder for Atmosphere) of ASI
- CARMEN-1 of CNES
The science data of these instruments are in cal/.val. phase. Many researchers are working on these issues and using the data. Most of them are not available for everybody yet, with the exception of the Aquarius data.
- The NIRST (New Infrared Sensor Technology) instrument of CONAE and CSA is still the commissioning phase.
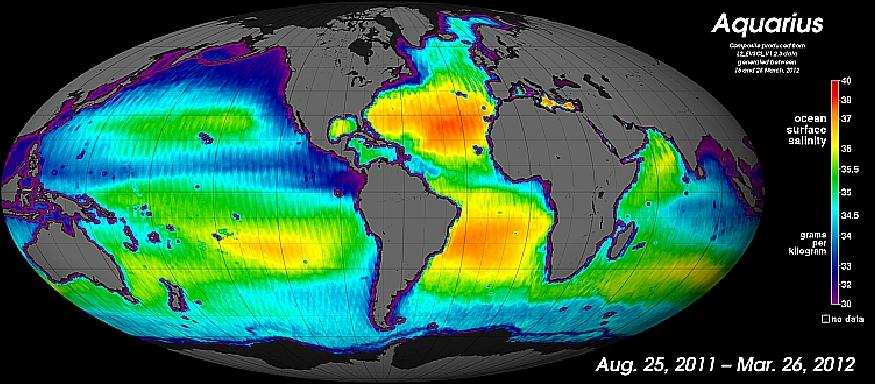
• December 1, 2011, marked the beginning of the Aquarius Science Operations Phase. The Aquarius sensor and the SAC-D observatory continue to perform well. During the holidays, the project reached a milestone of having received four-months of uninterrupted Aquarius data since the instrument commissioning sequence was finished on August 25, 2011. 35)
• During the months of October and November 2011, the project completed the Post Launch Assessment Review (PLAR) process with NASA.
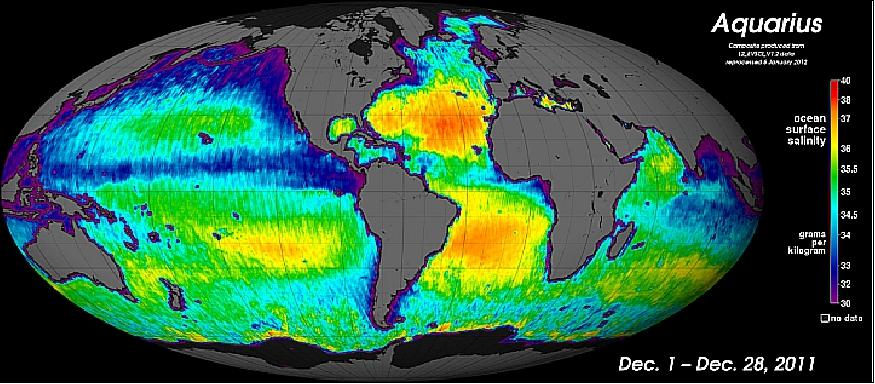
Legend to Figure 16: Sea surface salinity data taken by the NASA Aquarius instrument during one month. Reds show higher salinity (40 grams per kilogram) and purples show relatively low salinity (30 grams per kilogram). Preliminary (Version 1.2) data contain uncertainties and, over time, will be updated as further calibration and validation work are completed.
Data on this image in the southernmost ocean regions are not yet reliable as they are associated with high winds and low surface temperatures. The north-south striped patterns are artifacts of small residual calibration errors and thus are not real. Low salinity values immediately adjacent to land and ice-covered areas are due to proximity to coastlines or ice edges, which introduces errors into the data. The map shows several well-known ocean salinity features such as higher salinity in the subtropics; higher average salinity in the Atlantic Ocean compared to the Pacific and Indian oceans; and lower salinity in rainy belts near the equator, in the northernmost Pacific Ocean and elsewhere. These features are related to large-scale patterns of rainfall and evaporation over the ocean, river outflow and ocean circulation. 37)
• NASA released the first map of global ocean surface salinity in September 2011 (Figure 17). The new map is a composite of the first two and a half weeks of data since Aquarius became operational on August 25, 2011. The numerical values represent salt concentration in parts per thousand (grams of salt per kilogram of sea water). Yellow and red colors represent areas of higher salinity, with blues and purples indicating areas of lower salinity. Areas colored black are gaps in the data. The average salinity on the map is about 35. 38)
The map shows several well-known ocean salinity features such as higher salinity in the subtropics; higher average salinity in the Atlantic Ocean compared to the Pacific and Indian Oceans; and lower salinity in rainy belts near the equator, in the northernmost Pacific Ocean and elsewhere. These features are related to large-scale patterns of rainfall and evaporation over the ocean, river outflow and ocean circulation. Aquarius will monitor how these features change and study their link to climate and weather variations.
Other important regional features are evident, including a sharp contrast between the arid, high-salinity Arabian Sea west of the Indian subcontinent, and the low-salinity Bay of Bengal to the east, which is dominated by the Ganges River and south Asia monsoon rains. The data also show important smaller details, such as a larger-than-expected extent of low-salinity water associated with outflow from the Amazon River.
To produce the map, Aquarius scientists compared the early data with ocean surface salinity reference data. Although the early data contain some uncertainties, and months of additional calibration and validation work remain, scientists are impressed by the data's quality.
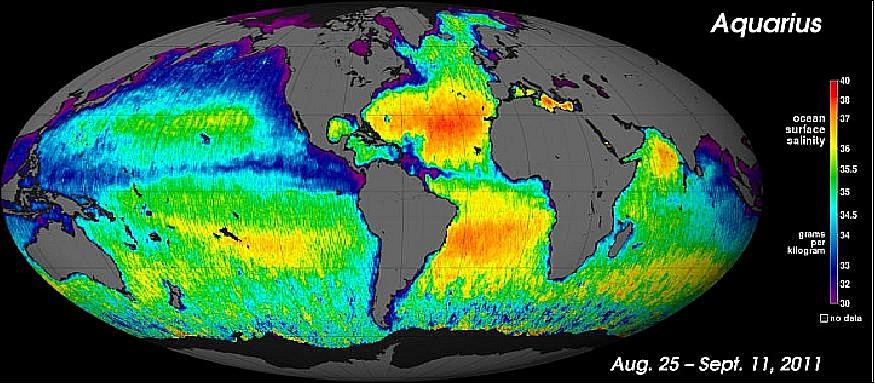
• Sept. 9, 2011: The Aquarius instrument has been operating in nominal science mode since August 25, with all radiometer and radar channels performing well. 40)
- Meanwhile, during the past two weeks the CONAE/SAC-D instrument team has been bringing all the other instruments on line. Preliminary results from the microwave radiometer (MWR) also look excellent. The other SAC-D instruments are still going through initial testing, and all have been powered-on successfully. At this time, the Aquarius calibration/validation (cal/val) working group has not detected any interference to Aquarius data related to the power-on of the SAC-D instruments, and this analysis is ongoing.
• The Aquarius instrument has successfully completed its initial commissioning phase and is now "tasting" the saltiness of Earth's ocean surface, making measurements from its perch in near-polar orbit.
- On Aug. 14, 2011, the Aquarius Instrument Flight Operations Team, together with the SAC-D Mission Flight Operations Team, began powering up the Aquarius instrument, and successfully completed deployment of the Aquarius antenna on Aug. 17, 2011. The team then began sequentially powering on the instrument's subsystems. On Aug. 20, the Aquarius radiometer, which collects the brightness temperature data from which salinity measurements are derived, was powered on for the first time in space and transmitted its first science data back to Earth, which were analyzed and found to be as expected. On Aug. 21, 2011, the team began powering on Aquarius' radar scatterometer, which corrects for the effects of ocean roughness on the radiometer readings. Commissioning of Aquarius was completed and regular data collection began on Aug. 24, 2011. 41)
- The Aquarius science team will spend the coming months analyzing and calibrating the measurements and releasing preliminary data.
- With the Aquarius instrument commissioning now complete, the SAC-D Instruments Flight Operations Teams, together with the SAC-D Mission Flight Operations Team in Argentina, are now engaged in commissioning the other seven SAC-D instruments. Once all the observatory instruments are commissioned, a maneuver will be conducted to place SAC-D / Aquarius spacecraft in its final orbit, 657 km above Earth (Ref. 41).
Question 1: Why wasn't it possible for the CONAE ops team, to commission the CONAE payloads while NASA was commissioning the Aquarius payload? Answer (provided by David M. Le Vine of GSFC): Besides being cautious (one thing at a time), the business of sending commands, verifying their execution and checking the data is time consuming and demanding of human resources. In addition, one goal of the Aquarius team was to confirm that there was no interference with the radiometer when the SAC-D instruments were turned on.
Questions 2: What was the orbital altitude during the commissioning phase? And why had the altitude to be different from the final altitude? Couldn't the S/C directly be deployed into its nominal orbit? Answer (David M. Le Vine): The launch was nearly perfect and the altitude was within a few 100 meters of the desired altitude. A small adjustment was made during the spacecraft commissioning that brought the altitude even closer to the desired 657 km. However, it was decided to postpone final tuning of the orbit so that instrument commissioning could begin sooner. The final tuning of the orbit is scheduled for September 12-October 3, 2011.
• With all observatory subsystems confirmed to be healthy, the SAC-D spacecraft commissioning activities were completed on July 24, 2011. The spacecraft's propulsion system then underwent a series of tests, and preliminary orbit adjustments were performed in preparation for turning on the observatory's eight science instruments.
• June 29, 2011: The SAD-D/Aquarius observatory commissioning phase is ongoing. It is anticipated that the Aquarius instrument will be turned on by the end of July. 42)
Sensor Complement: (Aquarius, NIRST, MWR, HSC, DCS, ROSA, CARMEN-1, TDP)
Instrument | Objectives | Specific measurements | Agency |
Aquarius (Microwave Radiometer & Scatterometer) | Understanding ocean circulation, global water cycle and climate interaction. Soil moisture over Argentina | Global sea salinity measurements & Retrieval of soil moisture from Aquarius over Argentina | NASA |
NIRST (New Infrared Sensor Technology) | Hot spot events, sea surface temperature measurements | Monitoring of hot spots caused by biomass fires and volcanic eruptions, estimation of fire radiative power Measurements of sea surface temperature (which also will enhance the results of Aquarius measurements) | CONAE, CSA |
MWR (Microwave Radiometer) | Precipitable water, winds speed, sea ice concentration, water vapor, cloud liquid water | Measurements of precipitable water and sea surface wind speed (which will enhance the results of Aquarius measurements). Measurements of water vapor, cloud liquid water and sea ice concentration | CONAE |
HSC (High Sensitivity Camera) | Urban lights, electric storms, polar regions, snow cover | Measurements of light intensity (urban areas, detection of electric storms, snow coverage, detection of fishing boats and aurora studies) | CONAE |
DCS (Data Collection System) | Collection of data from the ground segment | Collect environmental measured parameters (e.g. meteorological) | CONAE |
ROSA (Radio Occultation Sounder for Atmosphere) | Atmospheric properties | Measurements of atmospheric temperature, pressure profiles, and water vapor content | ASI |
CARMEN-1 (ICARE & SODAD) | Effects of cosmic radiation in electronic devices, distribution of micro-particles and space debris | Measure the high-energy radiation environment (ICARE). Measure the size distribution of micro- particles and space debris (SODAD) | CNES |
TDP (Technological Demonstration Package) | Technology demonstration | Position, velocity and time inertial angular velocity determination | CONAE |
Aquarius (Microwave Radiometer & Scatterometer)
The Aquarius instrument consists of an integrated pushbroom-type polarimetric microwave radiometer in L-band (passive device) provided by NASA/GSFC, and an S-band scatterometer (active device), designed and developed by NASA/JPL. The objective of the radiometer is to measure the microwave brightness temperatures of the ocean surface, which are sensitive to salinity and surface roughness. In parallel, the scatterometer measures the surface roughness for correcting the radiometric brightness temperature. The Aquarius radiometer/scatterometer instrument combination represents a first-of-its-kind instrument in a spaceborne mission. 44) 45) 46) 47)
The Aquarius science requirements call for a monthly global coverage of the oceans for the generation of sea-surface salinity maps. The baseline science data product will be on a grid of 150 km at < 0.2 psu (practical salinity unit) accuracy during the mission life of three years. In radiometric terms, a change of 1 psu corresponds roughly to a change in brightness temperature of about 0.5 K (the sensitivity depends on salinity, temperature, polarization and incidence angle). Hence, the goal in radiometric terms is on the order of 0.1 K. The mission will allow the observation of key seasonal and year-to-year SSS variations. To achieve its objectives the calibration stability requirement for the Aquarius radiometers is 0.15 K over two days. In-situ ground observations (buoys) will be conducted in parallel to spaceborne observations, to validate the observational data and to remove systematic errors in absolute sensor calibration.
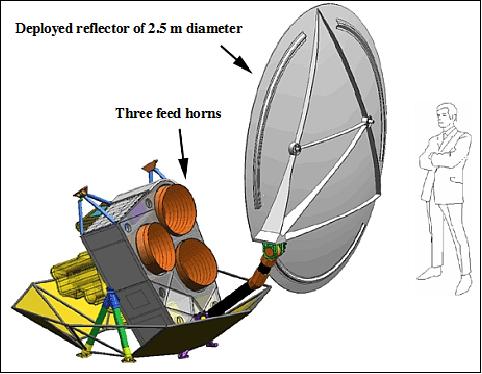
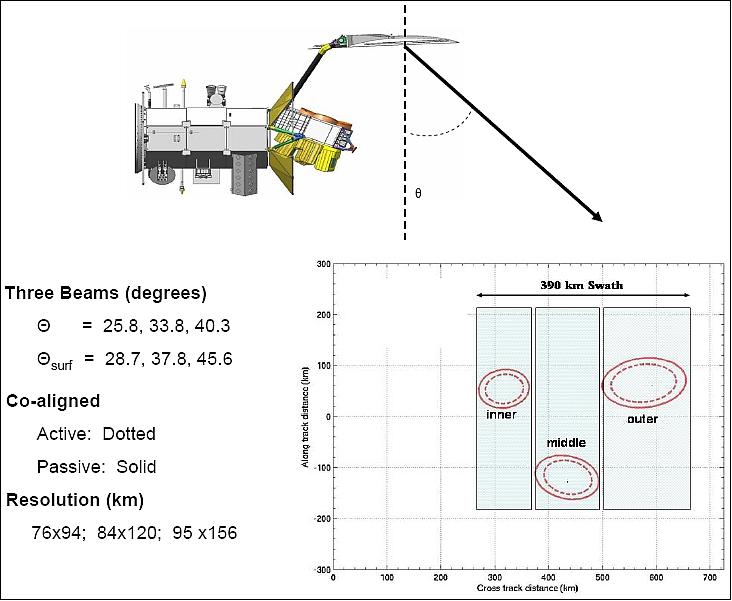
The Aquarius instrument views away from the sun to mitigate L-band solar flux from contaminating the Earth observation. This causes an asymmetry in the ground pattern over the poles, with the swath reaching to 88º N over the Arctic, and about 79.6º S over the Antarctic.
Sensor type | Radiometers (3) at 1.413 GHz |
Nr of channels | 3 antenna feeds, |
Scatterometer PRF, pulse width, calibration | 100 Hz, 0.98 ms, 0.1 dB |
Antenna aperture diameter (main reflector) | 2.5 m (offset parabolic reflector) |
Instrument size | 3 m x 6 m x 4 m, antenna deployed |
Aquarius instrument assembly | 375 kg |
Instrument power | 375 W duty cycle, 50 W standby |
Data rate | 5 kbit/s includes 20% contingency, 0.5 kbit/s standby |
Optical layout | 3 antenna beams at θ = 25.8º, 33.8º, 40.3º |
Footprint size of beams | 76 km x 94 km, 84 km x 120 km, 96 km x 156 km (total swath of about 390 km) |
Radiometer NEDT, 12 s integration | 0.05 K ; NEDT (Noise Equivalent Delta Temperature) |
Radiometer stability for 7 days | 0.12 K |
Radar (ALScat) calibration stability for 7 days | 0.13 dB |
Ground calibration scheme | In situ SSS sensors on buoys and ships |
On-orbit calibration scheme | Noise diodes in radiometer and cold sky measurements |
Pointing requirements (3σ) | 0.1º (knowledge); 0.5º (control and stability) |
Command and control requirements | Once per month for cold sky measurement |
Flight software architecture | Hybrid software |
Operational modes | ON, Standby, Survival |
Although Aquarius is a relatively "simple" pushbroom radiometer system (it only has 3 beams), it will demonstrate all the virtues of such a system once in space (apart from pixel numbers). The advantages of the pushbroom radiometer system (over a synthetic aperture radiometer system) are that it gives superior radiometric resolution and very likely also superior accuracy and stability. 48) 49) 50) 51)
ALRad (Aquarius L-band Radiometer) system. The overall objective is to measure SSS (Sea Surface Salinity). The radiometer is of polarimetric design with four separate channels and four noise diode calibration sources. A Wilkinson power divider provides an equal-power split for each radiometer channel. ALRad is of JMR (Jason Microwave Radiometer) heritage that uses a similar Dicke-switch radiometer with noise diode calibration. To achieve high calibration stability, ALRad employs a three-position Dicke switching method that provides nearly continuous calibration. The interface between ALRad and ALScat is via a diplexer - dividing the signal into two passbands. The diplexer provides a 60 dB isolation between ALRad and ALScat.
ALScat (Aquarius L-band Scatterometer) system. ALScat is a simple real-aperture radar without the requirement of pulse-to-pulse coherence. ALScat is a total-power dual-polarized L-band (1.26 GHz) slow-chirp system, designed to measure backscatter from the ocean surface. Its measurements will enable the removal of wind effects from the ALRad ocean-surface brightness temperature measurements being used to retrieve ocean salinity. The ALScat requirement calls for great stability with repeatability on the order of 0.1 dB over several days, and calibrated accuracy to this level over several months. The transmit signal waveform has a bandwidth of 4 MHz, a length of 1 ms, and is a frequency modulated chirp. The transmit power level is 200 W, providing a noise equivalent σo in the range of 0 dB to -40 dB (dynamic range). This is comparable to the expected cross-polarized scatterometer signal. 52)
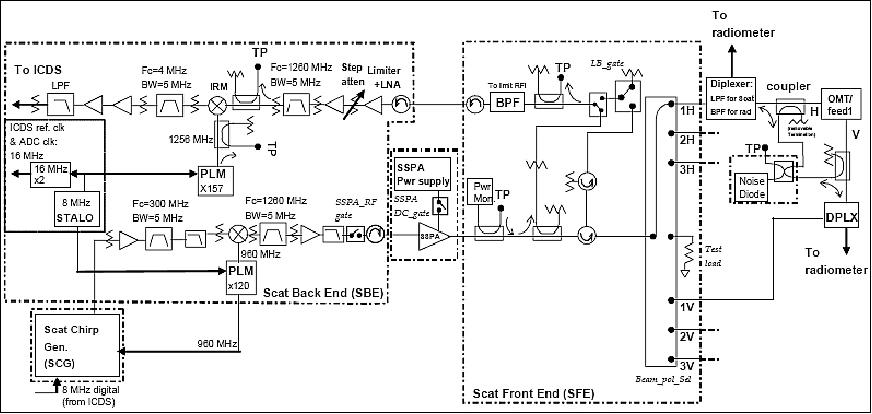
ALScat consists of SBE (Scatterometer Back End), SFE (Scatterometer Front End), as well as other diplexers, couplers, ortho-mode transducers (OMT), feeds, associated cabling, and the reflector. In addition, a chirp generator and SSPA (Solid-State Power Amplifier) are used to generate the high-power radar transmit signal. Digital data processing of the received signal is performed external to the Scatterometer subsystem by the ICDS (Instrument Command and Data Subsystem). ALScat will return estimates of backscatter power continuously over most of the Earth, including land and ice as well as ocean areas.
RFI (Radio Frequency Interference) considerations are important for the high sensitivity of the AlScat L-band receivers. The hardware for RFI detection aboard Aquarius is implemented in the digital portion of the radar receiver, using a radiation-hardened Actel RH1280 field-programmable gate array (FPGA). This FPGA was custom designed to include a digital square-law processor module and the RFI-threshold detection circuitry that flags periods of time where RFI contamination is suspected. This new element to the radar receiver makes it possible to identify and exclude, in ground data processing, those echoes that are likely to have RFI in the power measurement, before the science data is averaged further on the ground. 53) 54)
The ALRad system consists of several elements as shown in Figure 21. Beginning at the OMT (Orthomode Transducer), these elements are the OMT couplers, CND (Correlated Noise Diode), two diplexers (one for each polarization), the RFE (Radiometer Front End), the RBE (Radiometer Back End), and the DPU (Digital Processing Unit).
The feed structure consists of three feed-OMT assemblies mounted together near the focus of the reflector. The radiometer front end and associated electronics (diplexers that couple with the scatterometer, couplers for the correlated noise diode, etc) are mounted around the OMT and temperature controlled to provide a thermally stable environment for the critical RF components. The OMT assembly is cold-biased passively by means of radiator plates and appropriate coatings and actively controlled using heaters mounted to the assembly. The design requirement is to control the thermal environment to change less than 0.1º C over 7 days.
In the RFE, the two signals from the OMT (one for vertical polarization and one for horizontal polarization) are amplified and then combined to form four channels (vertical, horizontal and the sum and difference which is equivalent polarization at ±45º). The sum and difference signal is being used to compute the third Stoke's parameter (e.g. detected with a square-law detector in the RBE and later subtracted during the ground processing). The first elements at the input of the RFE are the Dicke switch and its reference load followed by a coupler to a noise diode that provides the hot load.
The RBE contains additional amplification, band-pass filtering, and the detectors for each channel. Its performance (stability) is less critical to the overall stability of the radiometer system because it is located behind all the calibration sources and after the first stage gain in the RFE. The design requirement for temperature control of the RBE is a maximum change of 0.4º C (rms) over 7 days.
The fundamental timing unit for the hardware is 10 ms (approximately 1 ms for the scatterometer transmit pulse and 9 ms of observation time for the radiometer). The ALRad and ALScat operation are alternated at a rapid rate so that the two sensors look at the same piece of ocean nearly simultaneously. The three radiometers (one for each beam) operate in parallel. During 120 ms each radiometer collects 7 samples (i.e. 9 ms long and repeated each 10 ms) looking into the antenna followed by 5 samples devoted to the calibration sources (two noise diodes and Dicke load). This 120 ms sequence is then repeated.
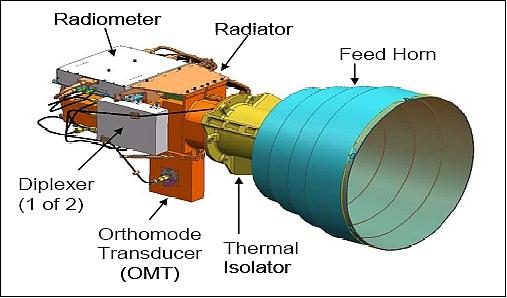
Antenna subsystem: The common payload antenna is a fixed offset system with three beams to scan the ocean surface. It employs a parabolic reflector (2.5 m diameter) illuminated by three feedhorns. The parabolic offset geometry eliminates feed blockage and leads to high main beam efficiency. A high beam efficiency is needed for calibration accuracy. On each of the feedhorns is an L-band radiometer (1.4 GHz) to measure ocean microwave emissions. An L-band (1.26 GHz) real-aperture scatterometer, ALScat, shares the antenna with the radiometer system.
AVDS (Aquarius Validation Data Segment): The AVDS will collect and process surface measurements for Aquarius data processing.
Platform | Quantity | Type | Distribution | Min. Depth | Latency |
Argo buoys | ~300/day | Profile float | Ocean-wide | 5 m | 1 day |
TAO/Triton | ~70 with salinity | Mooring | Tropical Pacific | 1 m | 1 day |
PIRATA | 15 | Mooring | Tropical Atlantic | 1 m | 1 day |
GDP buoys | Surface salinity TBD | Surface drifter | Ocean-wide | 1-2 m | 2 months |
Shipboard Thermosalinograph | varies | Hull-mounted | Ocean-wide | 5 m | 2 days |
GTSPP | varies | CTD profile | Ocean-wide | 1 m | TBD |
Background: The Aquarius heritage is of an airborne instrument called PALS (Passive and Active L- and S-band microwave instrument) of NASA (see Figure 22) - the objective was to study the use of multi-frequency, multi-polarization, passive and active data for remote sensing of coastal ocean salinity and soil moisture (time frame of PALS observations in 2002). Of the satellite sensors, there has been only one instrument that provides any heritage at L-band, namely the Skylab S-194 instrument (1.4 GHz, 124 km footprint diameter), a passive microwave radiometer that operated in the 1973/4 timeframe during the various Skylab missions. 55)
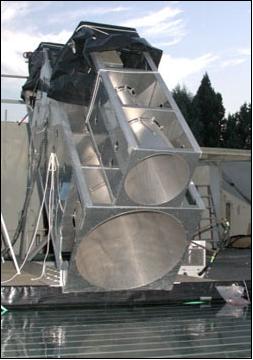
NIRST (New InfraRed Scanner Technology)
NIRST is an imaging multispectral radiometer, provided by CONAE and CSA (Canadian Space Agency). A cooperative agreement was signed between CONAE and CSA. The prime objectives are to detect high temperature thermal events (HTE) such as biomass burning and volcanic ash regions in the infrared spectral bands.
In this joint venture, CSA is providing the microbolometer detectors and camera electronics technology while CONAE's is providing two cameras in the MWIR (Midwave Infrared) and the TIR (Thermal Infrared) range. INO (Institut National d'Optique) of Quebec (Canada) is responsible for the development, the manufacturing and the verification of the camera module. The microbolometer sensors used in the camera module are developed jointly by INO and the CSA. Each camera is using one microbolometer of size 512 x 2 pixel arrays to scan the suborbital path in a pushbroom fashion. The combination of the MWIR and TIR bands allows for the retrieval of fire temperatures using the double band algorithm. Conversely the use of two TIR bands enables sea surface temperature measurements by means of the split algorithm technique. 56) 57) 58) 59)
The three band centers of the instrument are at 3.8 (MWIR), 10.85 and 11.85 µm (TIR); the resolution is 350 m on a swath width of 180 km. The instrument can be pointed in the cross-track direction about nadir providing a total FOR (Field of Regard) of 1064 km on a FOV of ±37.8º. The temperature range is, MWIR: 300-700 K and TIR: 250-500 K. NEDT: better than 0.5 K at 400 K in the MWIR range and better than 0.4 K at 300 K in the TIR range.
NIRST is a technology demonstration multispectral pushbroom imager. The radiometer is based on uncooled microbolometer detection. A 512 x 3 pixel microbolometer focal plane array (FPA) for multi-band pushbroom imaging has been developed. 60)
The NIRST instrument is housed in an aluminum container (35 cm x 40 cm x 50 cm in size) which is mounted on the nadir looking underside of the SAC-D spacecraft. The MWIR and TIR cameras are located next to each other in this compartment with their optical axis contained in a plane normal to nadir and normal to the satellite velocity vector.
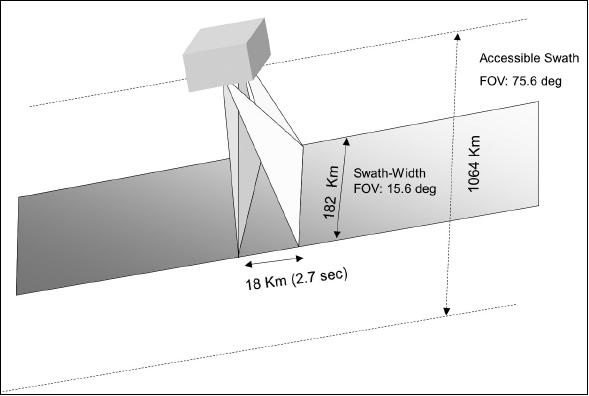
In view of improving the revisit time, NIRST is designed with a boresight pointing mechanism. To achieve a cross-track pointing capability, an optically flat, lightweight Beryllium mirror is used to steer the field of view of the cameras. The steering mirror can be rotated within a range from ±15º around its 45º position (nadir pointing).
The MWIR camera operates in the spectral band of 3.4-4.2 µm (band 1) and the TIR camera in the bands of 10.4-11.3 (band 2) and 11.4-12.3 µm (band 3). Each camera makes use of one array of 512 x 2 microbolometers. To achieve infield spectral separation, bandpass filters are mounted in front of one line of the MWIR array and on both lines of the TIR array. The MWIR and TIR cameras are optically aligned such that the ground pixel radiance can be co-registered in bands 1 and 2 at the same time. Registration in band 3 is slightly delayed with respect to that in bands 1 and 2 due to the physical separation between the two detector lines of the TIR camera.
Spectral band | MWIR | TIR1 | TIR2 |
Center wavelength | 3.8 µm | 10.85 µm | 11.85 µm |
Spectral range | 3.4-4.2 µm | 10.4-11.3 µm | 11.4-12.3 µm |
Temperature | 300 K (min) | 250 K (min) | |
NETD | < 0.5 K @ 400 K scene | < 0.3 K @ 300 K scene | < 0.4 K @ 300 K scene |
Temperature accuracy | 2.5 K @ 400 K scene | 1.5 K @ 300 K scene | < 2 K @ 300 K scene |
Ground pixel size (boresight pointing to nadir @ 657 km altitude) | 351 m |
Swath width (nadir) | 182 km |
In field separation (ground level) | 18 km |
Boresight pointing | ±30º from nadir |
FOR (Field of Regard) in cross-track | 1060 km |
The NIRST instrument makes use of two linear arrays of microbolometers, one optimized for operation in the MWIR band and the other for the two TIR bands. Each array comprises three components: a) Si micromachined bolometer pixels; b) readout integrated circuits (ROIC) monolithically built in the Si wafer; and c) radiometric package.
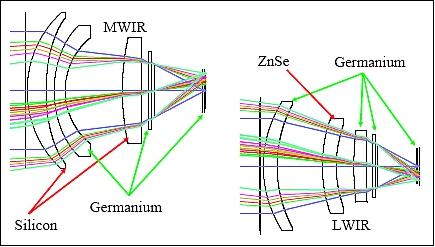
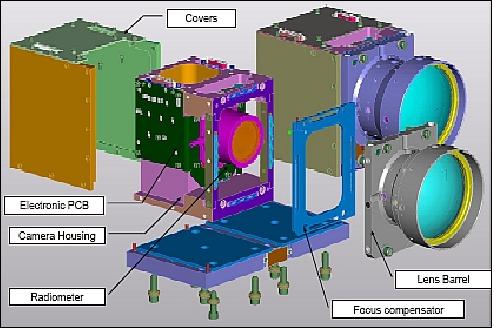
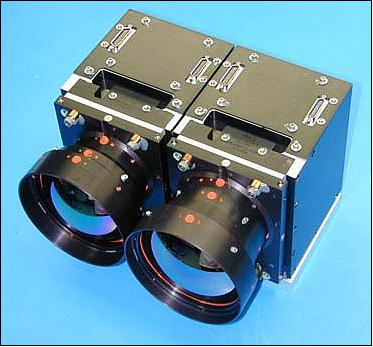
In-flight instrument operation: After the successful launch and post-launch verifications, the NIRST instrument started the acquisition protocol in the winter of 2012. To reach level 1 data, several data processing routines needed to be implemented, such as MWIR cross-talk correction and in-flight calibration protocol (Ref. 59).
The microbolometer array is built on a custom readout electronics that enables simultaneous integration of all pixels for scanning periods of up to 140 ms. Two arrays, integrated into two spectrally distinct radiometric packages, provide for coregistration of infrared images in three bands centered at 3.8, 10.85, and 11.85 µm for the retrieval of fire and sea surface temperatures.
Analysis of the downlinked data confirms the reliable in-orbit operation and consistency with prelaunch characteristics for both arrays. Algorithms have been developed to perform post processing and absolute radiometric calibration of images in all bands. Image deconvolution using Wiener filtering was found most effective in recovering the signal loss incurred in the active pixels when observing high temperature events. The in-flight gain and offset values were evaluated for all pixels by means of vicarious calibration with reference spaceborne sensors. Preliminary assessment of the images calibrated using these values showed that they are in agreement with those retrieved from GOES sensor. The MWIR high sensitivity to hot spot detection makes NIRST a useful tool for forest fires detection and monitoring.
MWR (Microwave Radiometer)
The MWR instrument is provided by CONAE. The objective is to provide measurements of surface brightness temperatures in the frequency range sensitive to geophysical parameters over the ocean such as: winds, rainfall, water vapor, and characteristics of sea ice. The MWR measurement geometry is designed to overlap Aquarius and contribute complementary science data for the SSS determination. 61) 62) 63) 64)
MWR is a 3-channel Dicke radiometer [23.8 GHz (500 MHz bandwidth, V polarization), and 36.5 GHz (1 GHz bandwidth, V and H polarizations] providing measurements with a resolution of 40 km. The antennas of MWR are arranged in pushbroom configuration. Each frequency is supported by a dedicated structural subsystem, consisting of a reflector, eight feed horns and two radiometers providing a swath width of 380 km, a resolution of 50 km and a sensitivity of 0.5 K, covering the same footprint as the Aquarius instrument.
- The MWR has a multi-beam configuration in each frequency
- The MWR shall be pointed towards the shadow side of the orbit to reduce the solar reflection and be coincident with Aquarius beams
- Elapse time between MWR and Aquarius observations < 150 seconds
- The MWR incidence angles shall be between 52º and 58º
- The brightness temperature stability: 1 K
- Same swath scene observation as Aquarius with an overlap of at least 95%
- The MWR instrument data and the ancillary data (e.g ephemeris, attitude and temperature, time) shall be time-tagged and delivered to the SAC-D Instrument Ground System for data processing.
- The MWR instrument has a mass of 99 kg and a power consumption of 96 W (orbital average power consumption).
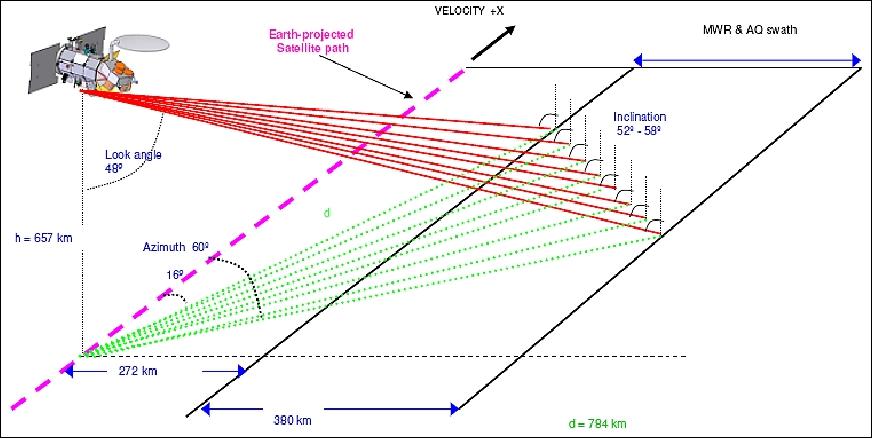
Instrument: MWR consists of two radiometer instruments operating at K-band and at Ka-band looking aft and forward, respectively, with regard to the flight direction. Each radiometer is connected to a set of eight feed-horns arranged in two rows of four feeds which illuminate a parabolic torus reflector as shown in Figure 28. The reflectors are scaled to match the 3dB beam width of the secondary antenna pattern at both the frequencies. The common 3db beamwidth is 1.64º, which results in an IFOV (Instantaneous Field of View) with a resolution of 50 km on the ground. Each row of feedhorn produces IFOV's lying on a conical arc on the ground.
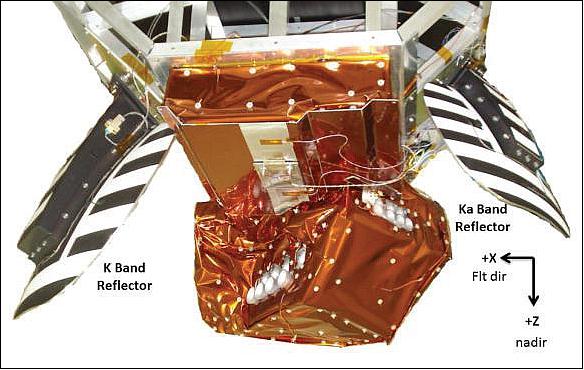
The left-hand side panel of Figure 29 depicts the conical geometry of the MWR beams. The IFOV's within a conical arc, closer to the subsatellite point along the radial direction, are at 52º incidence angle and those within the conical arc farther from the subsatellite point are at 58º incidence angle. The right hand side panel of Figure 29 shows the time progression of MWR IFOVs. The forward IFOVs at 36.5 GHz are marked in red and the aft IFOVs at 23.8 GHz are marked blue. It should be noted that there is a time lag between the forward and aft beams in sampling the same point on the Earth. This sensor geometry produces a swath width of 380 km, displaced 272 km across-track (towards the right), which exactly overlaps with the Aquarius instrument swath.
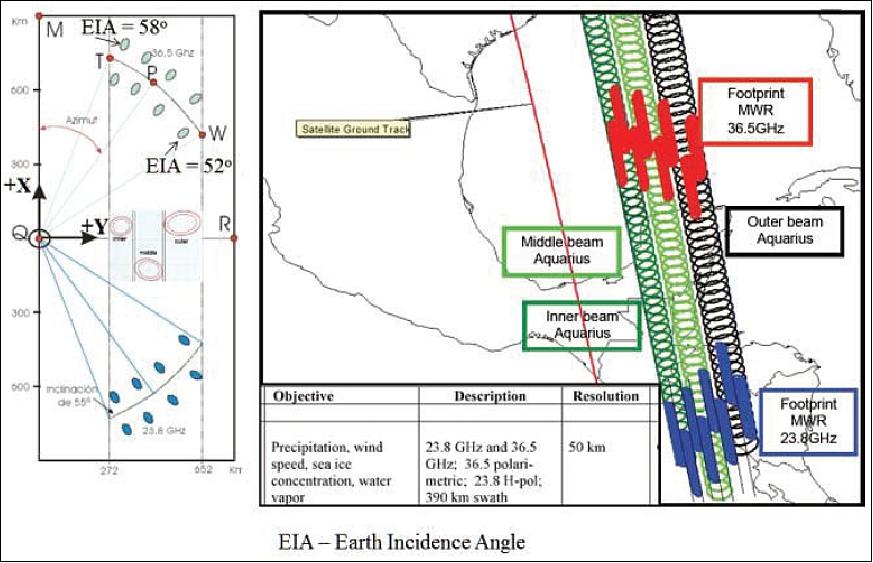
The MWR instrument measures four polarization states of the electromagnetic radiation at Ka-band. The horizontal (H) and vertical (V) polarization component of the signal from a Ka-band horn antenna is separated using an OMT (Ortho-Mode Transducer). After the OMT these two signal components are guided through two separate wave-guide paths and received by two separate radiometer receivers. The ±45º polarized channels are synthesized by cross-correlating the V and H polarized electric field signals using a hybrid coupler. Four separate detectors are used to detect all four polarimetric channels.
These measurements enable MWR to produce first three of the Stoke's parameters at Ka-band. Stoke's parameters are very useful in finding ocean surface wind direction and thus to correct for wind-direction induced wind-speed estimation error. For K-band measurements, there is only one radiometer receiver and one detector which only measure the H polarized component of the antenna signal. The K-band channel is mainly used to estimate the water vapor content of the atmosphere.
The whole MWR system consists of three Dicke type radiometers (one K- and two Ka-band channels), which calibrate themselves against gain fluctuations and thermal noise variations, by taking measurements of antenna temperature, noise (controlled) injected antenna temperature and a known reference temperature. For each of the radiometer three layers of switches are added at the front-end just after the OMTs to connect the eight feedhorns on a time-shared basis. The eight MWR beams of a given polarization are input to a single radiometer receiver. These beams are sampled sequentially with an integration time of 0.24 s as shown in Figure 30. The beam number indicates the feed horn at any time. The 0.24 s integration period is subdivided into eight subintervals of 30 ms duration and comprises an antenna measurement (S), an antenna plus noise measurement (S+N), and the reference load measurement (Tc). Thus 10 ms is allocated for taking each temperature measurement. During the last 1 ms of each of these 10 ms intervals, the Dicke switch connects the receiver to the reference load to protect the MWR from potential RFI (Radio Frequency Interference) produced by the Aquarius scatterometer transmit pulses. The 0.24 s integration time of each beam results in a beam sampling time of 1.92 s (i.e., each beam is sampled once each 1.92 s). At an altitude of 657 km, the SAC-D satellite travels with a velocity (Vs/c) of 7.53 km/s, which corresponds to a ground velocity (Vg) of 6.82 km/s. At this ground velocity, in 1.92 s beam sampling time, the beams travel an along-track distance of 13.1 km. Thus, MWR footprints have 70% overlap in consecutive along-track sampling (considering a 50 km footprint size). However, in the cross-track direction, there is no overlap and the cross-track resolution is limited by the footprint size.
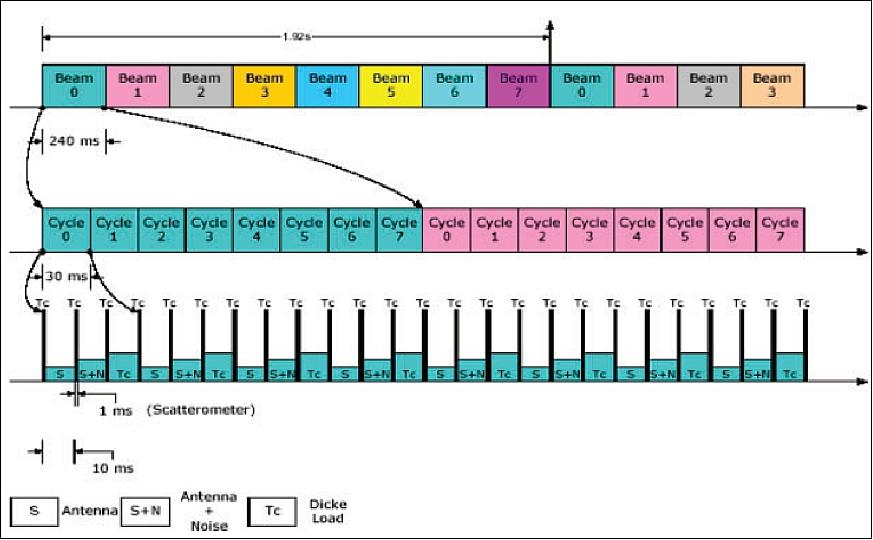
Receiver calibration: The total radiation brightness (Tap) captured by any particular antenna feed horn under observation is guided to the receiver through a switching network. The feed-horn and all successive components in the path of the signal from antenna to the receiver have finite losses and corresponding self-emissions, which modifies Tap. Also the reflections at the horn aperture and leakage through the switches modify the input/output relationship of the radiative transfer. The modified Tap at the receiver input (Tin) could be related to the radiometer counts using equation (1) in Figure 31.
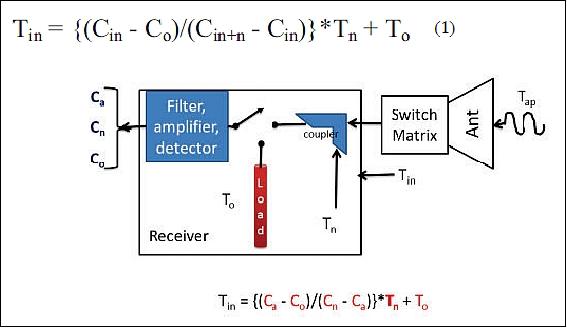
Where, Cin, Cin+n and Co are the radiometer output counts due to Tin, Tin + injected noise and reference load respectively. The reference load temperature To, is obtained in real-time from MWR's internal temperature sensors. The injected noise temperature Tn, is measured in the laboratory using external calibration loads before launch, and the assumption is it's stable during the flight.
Coriolis/WindSat mission comparison
Coriolis/WindSat (of NRL, launch Jan. 6, 2003) and MWR share similarities in orbit ground track and radiometric frequencies, enabling the possibility of obtaining a large number of collocated radiometric measurements which could be easily compared. Also, the objective of WindSat mission is to investigate the use of microwave radiometers and to measure both wind speed and direction over the ocean; this is extremely demanding on the quality of the calibration and the sensor had commensurate care applied to its calibration.
The MWR was powered on after 81 days from SAC-D launch on August 30, 2011. The first Tb images were produced using the pre-launch algorithm (v2.0) ~ 6 hours after the data reception on August 31, 2011. The v2.0 algorithm is based on the analysis of the T/V data and as mentioned earlier, it gives an estimate of the antenna temperature (Ta) but not the actual main beam brightness temperature (Tb). Thus a comparison of the MWR Ta with WindSat Tb is performed after gathering 65 MWR orbits. The MWR Ta bias with respect to WindSat Tb is modeled as a linear function of MWR Ta. This enabled the project to determine a slope-offset correction similar to APC, which is used to obtain final MWR Tb.
A comparison between the MWR bias histograms using pre-launch (v2.0) and the improved post-launch (v2.2) calibration algorithm is shown in Figure 32, for the 36.5 GHz H-polarized channel. It should be noted that the v2.0 bias histograms (marked with dashed lines) are having non-zero mean and a small slope error (which makes them deviate from ideal Gaussian shape). Whereas, after the slope/offset correction, the v2.2 bias histograms (solid line) are zero mean and of Gaussian shape. Also the biases for all the beams are balanced with respect to each other. The MWR inter-beam biases are found to be negligible in comparison with WindSat.
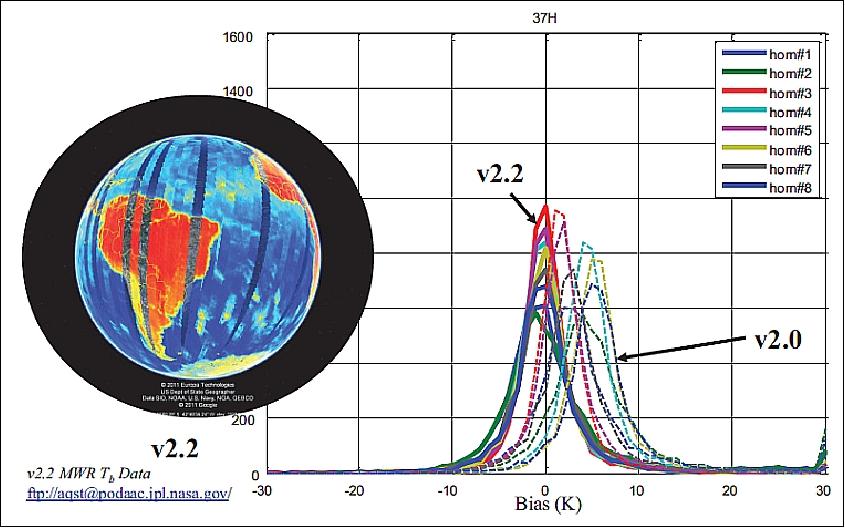
HSC (High Sensitivity Camera)
The HSC instrument is provided by CONAE. HSC is a panchromatic camera (450-900 nm) providing a spatial resolution of 200-300 m on a swath of ± 700 km about nadir. The objective is to detect electric storms, urban lighting, fires and aurora events at night. The camera is based in TDI CCD technology. A similar camera is already on board of SAC-C satellite, obtaining images since its launch in November 2000.
DCS (Data Collection System)
The DCS is provided by CONAE. The objective is to collect environmental data of DCPs (Data Collection Platforms) in the prevailing ground segment. The data are later being transmitted to the Cordoba ground station and to users via the Internet. The ground platforms (DCPs), at least 200, have sensors of meteorological and environmental parameters. 65)
Although the DCS is intended to provide a national (Argentina) and regional coverage, with a footprint of 2300 km in diameter, the messages of the DCS are completely Argos system compatible. The uplink operates in the UHF band at 401.55 MHz.
ROSA (Radio Occultation Sounder for the Atmosphere)
ROSA is a GPS occultation receiver of ASI (Agenzia Spaziale Italiana), the Italian Space Agency. ROSA has been designed and developed at TAS-I (Thales Alenia Space-Italia). The instrument is also being flown on the OceanSat-2 mission of ISRO (a launch of OceanSat-2 is planned for 2009). ROSA measures the atmospheric (troposphere and stratosphere) temperature and humidity profiles using the GPS Radio Occultation measurement technique. 66) 67)
The receiver measures the bending angle between the direction of the undisturbed radio signal and the apparent incoming direction of the signal. The main objectives of the program are to test and to verify the capability of ROSA for the following applications:
• Meteorology/ Climatology (temperature and humidity profiles)
• Space weather (electron density profiles in the ionosphere), retrieval of TEC (Total Electron Count)
• Solid Earth physics (POD).
The major elements of the ROSA instrument are:
• GPS receiver for RO (Radio Occultation)
• Velocity & anti-velocity antenna assembly
• POD (Precise Orbit Determination) antenna
• RF cables connecting antennas with receiver.
Receiver type | Integrated GPS receiver for atmospheric sounding |
Channels | 16 dual frequency channels (equal to 48 single channels) |
Frequency band | GPS L1: 1575.42 MHz |
Observables | L1CA, L1P & L2P code phase |
Real-time orbit determination | Use of GPS C/A signal observations and navigation messages (PVT solution through SPS and Navigation Kalman Filter) |
ROSA is flown on-board of the following three missions: 68)
1) OceanSat-2 of ISRO, launched on Sept. 23, 2009.
2) SAC-D of CONAE and NASA, launched on June 10, 2011.
3) Megha Tropiques of ISRO and CNES, launched on Oct. 12, 2011.
For the SAD-D installation, TAS-I delivered 2 Lagrange Navigation Rx and a ROSA instrument. Strong pulsed interference from the Aquarius instrument required a dedicated RF filter box. In this configuration, ROSA has velocity and anti-velocity antennas that allow rising and setting occultations with a FOV of ±45°.
ROSA instrument: The ROSA receiver is a GPS receiver for spaceborne applications, specifically conceived for atmospheric sounding by radio occultation, which is able to determine position, velocity and time using GPS signals. Besides providing real-time navigation data, ROSA is able to accurately measure pseudoranges and integrated carrier phase (raw data), to be later processed on ground for scientific purposes. ROSA processes the received GPS signals in both the L1 and L2 frequency bands, allowing compensation of ionospheric delays. A codeless tracking scheme is included, in order to process the encrypted P(Y) signals transmitted in the L2 frequency band. The ROSA instrument, in its complete configuration, is composed by the follow parts (Ref. 68):
• One navigation antenna to acquire the GPS signals to determine position, velocity and time of the object where there is the receiver.
• Two radio occultation antennas to acquire the GPS signals used in the calculus of all the parameters used in the atmospheric sounding (for complete instrument). The Oceansat-2 tailored version features only the velocity antenna.
• RF (Radio Frequency) cables connecting the antennas (for navigation and radio occultation) to the receiver box.
• Receiver box which processes the GPS signals from all the antennas.
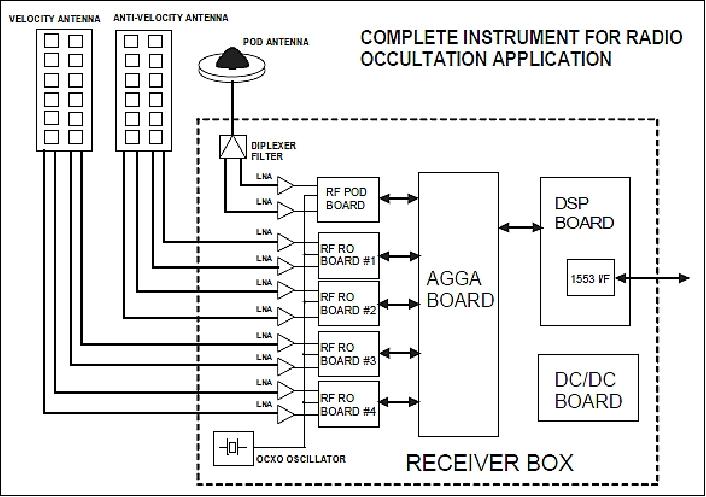
Legend to Figure 33: The OceanSat-2 tailoring features only one occultation antenna (the velocity antenna).
The instrument is equipped with one hemispherical-coverage antenna that is mounted with boresight direction equal to the zenith direction and is used to track the GPS signals for navigation purpose and for POD (Precise Orbit Determination). In addition, one directive velocity antennas is mounted on the OceanSat-2 spacecraft. This antenna is oriented in such a way to be able to track signals from GPS satellites in Earth occultation. Sixteen dual-frequency channels (4 AGGA chips) are available in the ROSA Receiver, and can be freely assigned to any combination of satellites.
ROSA is provided with a MIL-STD-1553 communication interface over which telecommand, telemetry and measurement data are exchanged. The receiver digital section is based on an ADSP 21020 processor and four AGGA-2a channels ASIC. In summary, the ROSA receiver performs the following main operations:
• Receives L1 C/A, L1 P(Y) and L2 P(Y) signals from GPS satellites and automatically allocates HW channels to GPS satellites.
• Obtains and maintains code lock and carrier lock, demodulates and decodes data message and recovers navigation data from each received GPS satellite, in navigation and occultation, the latter using a robust PLL (Phase Locked Loop) aided by a FLL (Frequency Locked Loop).
• When at least 4 GPS satellites are in view, performs position, time and velocity calculation based on a least square algorithm. In parallel, it performs Kalman filtered solutions.
• Uses calculated position information to establish geometrical line of sight information of each acquired GPS satellite with respect to the receiver platform and maintains a tracking list of visible satellites.
• Performs autonomous forthcoming occultation event prediction and channel allocation.
• When carrier lock is not possible, performs open loop high-rate sampling of raw observables (I, Q) for carrier phase reconstruction on ground.
• Provides observable data for each GPS satellite in lock.
• Monitors and maintains receiver health status.
• Allows receiver control from ground through user commands and provides telemetry data.
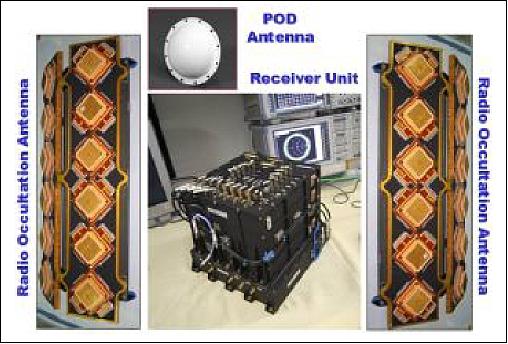
The antenna for navigation (used also for POD) is a dual-band L1/L2, with hemispherical coverage pattern; the gain is greater than – 4 dBic for elevations > 5° and RHCP (Right Hand Circular Polarization). The ROSA radio occultation antenna is of critical importance due to the fact that its scope is to receive the strongly attenuated signals coming from the GPS constellation during their occultation time window. The electromagnetic signals passing through the low atmosphere during the satellite occultation phase are subject to increased propagation losses with respect to the navigation antenna atmospheric losses.
The antenna is connected to the ROSA receiver unit by two different RF cables for L1 and L2 frequency signals directly to the LNA's, without passing through the diplexer used for the navigation one. Two BPF (Band-Pass Filter) boxes are mechanically attached to the antenna, centered around the GPS carriers L1/L2.
CARMEN-1
CARMEN-1 = ICARE-NG+SODAD (Influence of Space Radiation on Advanced Components- New Generation) of CNES, France. ICARE is a radiation monitoring instrument (in situ measurements of electron, proton and ion fluxes) while SODAD (Système orbital pour la détection active des débris) is a orbital debris/micrometeorite/µparticle detector. ICARE was first flown on the SAC-C mission of CONAE (launch Nov. 21, 2000 and still operational as of 2007). 69)
The ICARE objectives are to support:
• The improvement of risk estimation models for radiation effects on the latest generations of IC technologies
• Updates of environment models for radiation responsible for electronic component degradations and breakdowns
• Real-time monitoring of environmental conditions for preventive measures.
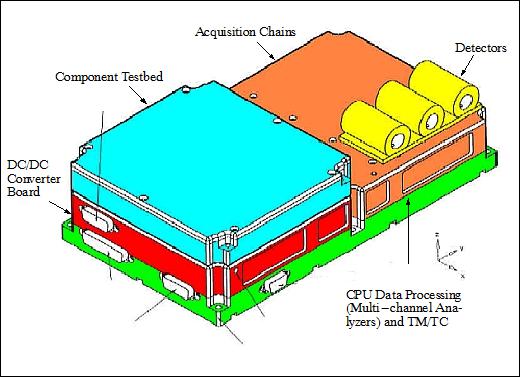
ICARE consists of the following elements: particle detectors, acquisition electronics, processing unit (CPU), bus interface unit (MIL-STD.-1553 controller), and a power supply. The component testbed is realized as an independent test module. The instrument has a mass of 2.4 kg, a mean power consumption of 2.5 W and a size of 281 mm x 155 mm x 71 mm. A description of ICARE is presented under SAC-C. - ICARE-NG (Next Generation) is a redesign of ICARE (ONERA) with about half the volume (116 mm x 202 mm x 90 mm) and a mass of 2 kg.
The objectives of SODAD are to perform "clouds" analysis; compare results with laboratory tests and numerical simulations; understand kinetics of space damage and to evaluate orbit population and its evolution.
TDP (Technological Demonstration Package)
TDP is provided by CONAE with the objective to test a new design for POD (Precise Orbit Determination) applications of future CONAE missions. The instrument is composed of two units:
1) IRU (Inertial Reference Unit) providing spacecraft triaxial inertial angular velocity in the range of 10º/s to 0.5º/s with an angular random walk (ARW) of 0.008º/sqrt hr.
2) GPSR (GPS Receiver) which provides the parameters: spacecraft position, linear velocity, time and pulse per second (PPS) with errors of 20 m in 3-D (1σ), real time and < 10 m post facto, linear velocity 1 m/s (1σ), and < 0.2 µs (1σ).
Project Implementation
The two projects, Aquarius and SAC-D, have a MOU-based agreement to establish, for the entire life-cycle of the mission, a joint set of requirements, policies and documents that define the basis of formulation, implementation and operation - The Aquarius/SAC-D Project Implementation Plan (PIP). Similar Letter of Agreements (LOAs) and MOUs have been established by CONAE with ASI, CNES, AEB (Agência Espacial Brasileira), and CSA.
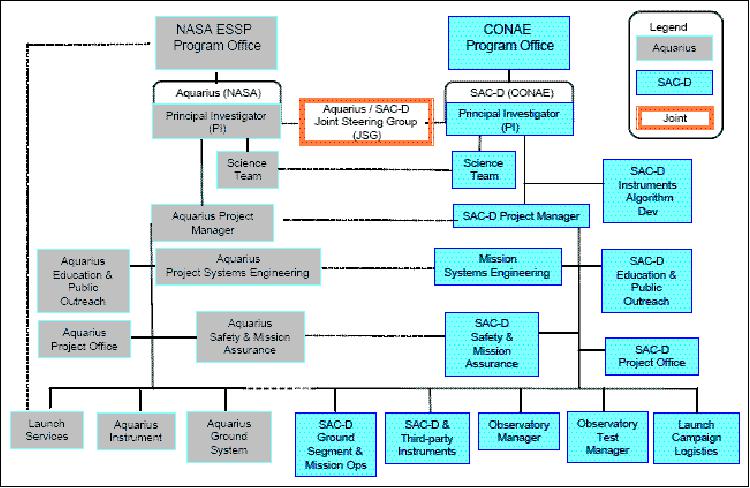
The requirements call for the integration of the sensor complement (Aquarius, CONAE, and the 3rd party instruments) onto the SAC-D platform at the INVAP facility in Bariloche, Argentina. Upon completion of observatory integration, the SAC-D/Aquarius observatory will then be transported to the INPE/LIT (Instituto Nacional de Pesquisas Espaciais / Laboratório de Integração e Testes) facility in Sao Jose dos Campos, Brazil for observatory-level environmental testing. Upon completion of the environmental campaign, the observatory will be shipped by air-transport to VAFB (Vandenberg Air Force Base), CA, for integration to the launch vehicle and finally launch in July 2009. After launch, the observatory will perform a 45 day IOC (In-Orbit Checkout). Completing the IOC phase will start routine science operations for a projected operational lifetime of 5 years.
Ground Segment
The CONAE MOC (Mission Operations Center), located at the Teófilo Tabanera Space Center in the Province of Córdoba (Argentina), is responsible for planning, commanding and monitoring the Argentine satellites. The primary ground station of SAC-D/Aquarius is the ETC (Estación Terrena Córdoba - or Cordoba Ground Station). ETC is located at 31° 31' South and 64° 27' West. The ETC coverage makes data acquisition possible form Argentina, Chile, Bolivia, Paraguay, Uruguay, a large part of Peru and the south of Brazil.
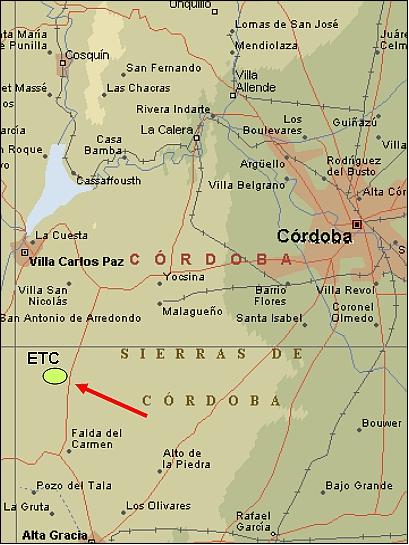
Surrounded by the Cordoba hills,CONAE's Teofilo Tabanera Space Center (CETT) is located in the Falda del Carmen region, close to the city of Alta Gracia. Its Ground Station receives information produced by more than 16 Earth observation satellites from different countries, such as Landsat, ERS, Terra, Aqua, SPOT and the RADARSAT missions. The station also provides the crucial service of tracking, monitoring and control of the Argentinean satellites SAC-C and SAC-D/Aquarius and of the Italian COSMO-SkyMed constellation.
NASA provides additional ground stations (3) during LEOP (Launch and Early Operations Phase - including spacecraft positioning and calibration), and during any serious contingencies using NEN (Near Earth Network) , formerly referred to as NGN (NASA Ground Network). The NASA ground stations are located in Svalbard, Norway (Spitzbergen); Poker Flats, Alaska; Wallops, Virginia, and McMurdo, Antarctica (Ref. 10). 70)
During nominal operations, Aquarius data will be sent from the MOC to the GSFC for processing where salinity products will be produced.
CONAE has agreed with ASI to support the SAC-D/Aquarius mission using its ground stations at Malindi, Kenya and Matera, Italy. The Malindi station will provide S-band downlink capability during critical launch activities including launch vehicle separation and solar array deployment. The Matera station will be used throughout the mission to augment the CONAE station for maintaining robust X-band downlink of science and mass memory playback data.
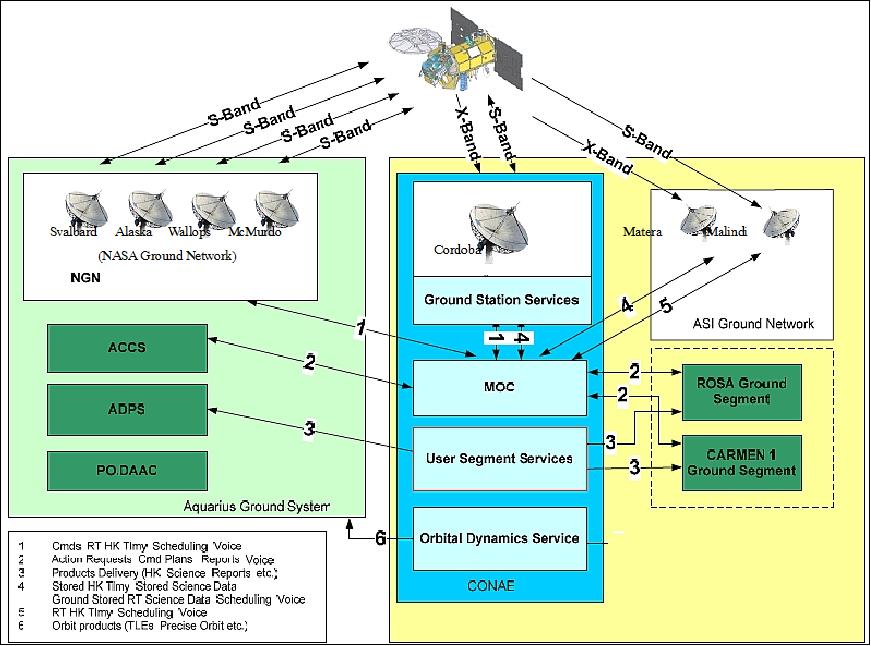
The MOC is provided by CONAE which is responsible for mission operations management. The MOC architecture is an evolution of the proven design currently supporting SAC-C. The MOC provides the coordination between CONAE's RF station service, the NGN stations and the ASI ground network. The MOC coordinates with the instrument user ground segments for instrument operations and data retrieval. The main functions of the MOC include:
• Mission operations planning and design
• Science and instrument operations support
• Command planning, verification and uploads (including testing of commands on the observatory engineering model)
• Ground station interaction (CONAE, ASI and NGN ground stations)
• Observatory health monitoring and housekeeping trending
• Observatory navigation and orbit maintenance maneuver implementation.
The Aquarius ground segment is built on a proven set of infrastructure and experience that is currently supporting SeaWiFS, MODIS/AQUA, MODIS/Terra along with other missions. The system functions are divided into three areas: instrument command and control, science data processing, archival & distribution, and NASA ground station resource coordination.
The Aquarius data processing segment provides for a highly automated data system for acquiring, processing, analyzing, archiving and distributing scientific data. The ADPS (Aquarius Data Processing Segment) automatically retrieves raw downlink files of Aquarius science data from the CONAE database and processes this data into time-ordered salinity data files. A month's worth of these files are processed with ancillary instrument and Observatory housekeeping data to produce a single sea surface salinity map.
Operating and monitoring the Aquarius Instrument's health is the function of the ACCS (Aquarius Command and Control Segment). The ACCS receives the instrument housekeeping data along with ancillary Observatory data necessary to assess the instrument's health and status. The ACCS coordinates with the Aquarius Science Team and the SAC-D Operations personnel to plan instrument activities and command sequences. The ACCS leads the resolution of any instrument anomalies, again in coordination with the Science Team and the SAC-D MOC. Each Aquarius instrument command activity, nominal or contingency, is verified using the Aquarius testbed.
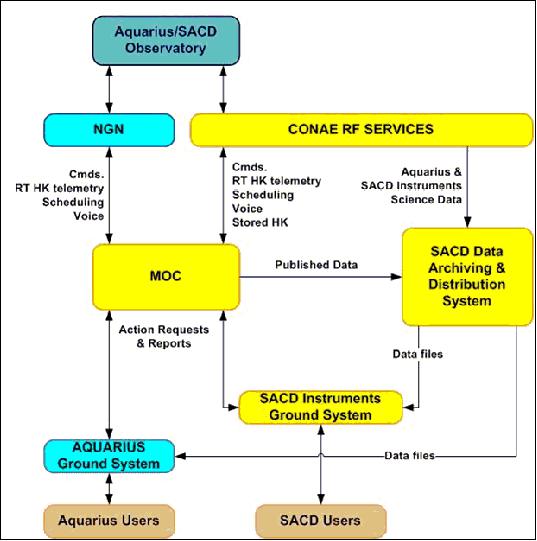
References
1) G. S. E. Lagerloef, Y. Chao, F. R. Colomb, "Aquarius/SAC-D Ocean Salinity Mission Science Overview," Proceedings of IGARSS 2006 and 27th Canadian Symposium on Remote Sensing, Denver CO, USA, July 31-Aug. 4, 2006
2) D. M. Le Vine, G. S. E. Lagerloef, S. Yueh, F. Pellerano, E. Dinnat, F. Wentz, "Aquarius Mission Technical Overview," Proceedings of IGARSS 2006 and 27th Canadian Symposium on Remote Sensing, Denver CO, USA, July 31-Aug. 4, 2006
3) G. Lagerloef, R. F. Colomb, D. Le Vine, Yi Chao, "Aquarius/SAC-D, Mission Status: Sea Surface Salinity, Understanding the Interactions Between the Global Water Cycle, Ocean Circulation and Climate, Aquarius Validation Data Segment," 6th SMOS Workshop, Copenhagen, Denmark , May 15-17, 2006
4) G. Lagerloef, R. F. Colomb, D. Le Vine, Yi Chao, "Aquarius/SAC-D, Sea Surface Salinity, Understanding the Interactions Between the Global Water Cycle, Ocean Circulation and Climate," 5th International SMOS Workshop: Nov. 29 - Dec. 1, 2004, ESA-ESRIN, Frascati, Italy, URL: http://earth.esa.int/workshops/smos04/presentations/SMOS_SW5_Aquarius_status.pdf
5) E. F. Stocker, C. Koblinsky, "Sea-surface salinity: the missing measurement," Proceedings of SPIE, Vol. 4881, Crete, Greece, Sept. 23-27, 2002
6) S. H. Yueh, W. J. Wilson, E. Njoku, D. Hunter, K. S. Kona, K. Bahadori, Y. Rahmat-Samii, "Compact, Lightweight Dual-Frequency Microstrip Antenna Feed for Future Soil Moisture and Sea Surface Salinity Missions," Proceedings of ESTC (Earth Science Technology Conference), College Park, MD, June 24-26, 2003
8) G. Lagerloef, J. Font, "Aquarius SAC-D Mission Overview," SMOS 4th Science Workshop, Porto, Portugal, April 2003, URL: http://www.cesbio.ups-tlse.fr/data_all/SMOS_WS/WS4/7-AquariusSAcD.pdf
9) A. Sen, Y. Kim, D. Caruso, G. Lagerloef, R. Colomb, S. Yueh, D. Le Vine, "Aquarius/SAC-D Mission Overview," Proceedings of SPIE, "Sensors, Systems, and Next-Generation Satellites X," edited by Roland Meynart, Steven P. Neeck, Haruhisa Shimoda, SPIE Vol. 6361, Stockholm, Sweden, Sept. 11-14, 2006, doi: 10.1117/12.691981
10) Gary Lagerloef, F. Raul Colomb, David Le Vine, Frank Wentz, Simon Yueh, Christopher Ruf, Jonathan Lilly, John Gunn, Yi Chao, Anette Decharon, Gene Feldman, Calvin Swift, "The Aquarius/SAC-D Mission - Designed to meet the Salinity Remote-Sensing Challenge," Oceanography, Vol. 21, No 1, March 2008, pp. 68-81, URL: http://www.tos.org/oceanography/issues/issue_archive/issue_pdfs/21_1/21.1_lagerloef.pdf
11) Gary Lagerloef, Aquarius/SAC-D Mission - Mission Update and Overview," 5th Science Meeting, Buenos Aires, Argentina, Oct. 21-23, 2009, URL: http://www.conae.gov.ar/AQ_SAC-D_5thScienceMeet/3_G_Lagerloef.pdf
12) Amit Sen, Daniel Caruso, David Durham, Carlos Falcon, "Aquarius/SAC-D: An International Remote Sensing Satellite Mission measuring Sea Surface Salinity," Proceedings of the SPIE Remote Sensing Conference, Toulouse, France, Vol. 7826, Sept. 20-23, 2010, paper: 7826-27, 'Sensors, Systems, and Next-Generation Satellites XIV,' edited by Roland Meynart, Steven P. Neeck, Haruhisa Shimoda, doi: 10.1117/12.866352
13) CONAE-NASA, Joint Process to Select an International Science Investigating Team for the Aquarius/SAC-D Observatory," URL: http://www.conae.gov.ar/SAC-D_AQ_AO/JointProcess_SACD-AQ_AO_CONAE-NASA_v2.pdf
14) F. R. Colomb, G. S. E. Lagerloef, D. Le Vine, I. Nollmann, Yi Chao, "The SAC-D/Aquarius Mission, Scientific Objectives," Gayana (Concepción), 2004, http://www.scielo.cl/scielo.php?pid=S0717-65382004000200022&script=sci_arttext#t1
15) A. Sen, Y. Kim, D. Caruso, G. Lagerloef, R. Colomb, S. Yueh, D. Le Vine, "Aquarius/SAC-D Mission Overview," Proceedings of SPIE, "Sensors, Systems, and Next-Generation Satellites X," edited by Roland Meynart, Steven P. Neeck, Haruhisa Shimoda, SPIE Vol. 6361, Stockholm, Sweden, Sept. 11-14, 2006, doi: 10.1117/12.691981
16) G. S. E. Lagerloef, D. M. Le Vine, Y. Chao, R. Colomb, I. Nollman, "Global monitoring of Sea Surface Salinity with Aquarius," URL: http://ursiweb.intec.ugent.be/Proceedings/ProcGA05/pdf/F06.3(01037).pdf
17) http://www.invap.com.ar/en/goverment-and-aerospace-area/projects/sac-daquarius-satellite.html
18) Information provided by Raul Colomb and Ida Nollmann of CONAE, Buenos Aires, Argentina
19) Sandra Torrusio, "Aquarius/SAC-D Observatory Mission Overview," April 12-16, 2010, URL: http://www.mincyt.gob.ar/multimedia/archivo/archivos/Sandra_Torrusio.pdf
20) "NASA's 'Age of Aquarius' Dawns with Launch from California," NASA, June 10, 2011, URL: http://aquarius.nasa.gov/pdfs/11-181_Aquarius_Launch_SC.pdf
21) "Ocean-watching satellite facing delays in Argentina," Spaceflight Now, Dec. 26, 2009, URL: http://www.spaceflightnow.com/news/n0912/26aquarius/
22) Sandra Torrusio, Daniel Caruso, Amit Sen, "Aquarius/SAC-D Mission Update," 6th Aquarius/SAC-D Science Meeting, Seattle, WA, USA, July 19-21, 2010
23) Alan Buis, Dwayne Brown, Rani Gran, "International Spacecraft Carrying NASA's Aquarius Instrument Ends Operations," NASA/JPL News Release 2015-207, June 17, 2015, URL: http://www.jpl.nasa.gov/news/news.php?feature=4628
24) "2014 Aquarius / SAC-D Science Team Meeting," Nov. 11-14, 2014, Seattle, WA, USA, URL: http://depts.washington.edu/uwconf/wordpress/aquarius/
25) Gary Lagerloef, "Aquarius Satellite Salinity Measurement Mission Status, and Science Results from the initial 3-Year Prime Mission," 2014 Aquarius/SAC-D Science Team Meeting, Nov. 11-14, 2014, Seattle, WA, USA
26) Gary Lagerloef, David Le Vine, Hsun-Ying Kao, "Aquarius salinity data validation and science status," Proceedings of IGARSS (IEEE Geoscience and Remote Sensing Society) 2014, Québec, Canada, July 13-18, 2014
27) Maria-José Viñas, "NASA's Aquarius Returns Global Maps of Soil Moisture," NASA, July 7, 2014, URL: http://www.nasa.gov/content/goddard/nasas-aquarius-returns-global-maps-of-soil-moisture/#.U7rWNZWevpY
28) Annette deCharon, David Le Vine, "An Update on the Aquarius Mission: Two-and-a-Half Years and Going Strong," The Earth Observer, NASA, Jan.-Feb. 2014, pp: 5-11, URL: http://eospso.gsfc.nasa.gov/sites/default/files/eo_pdfs/Jan-Feb_2014_final_color_508.pdf
29) Gary Lagerloef, "Mission Status & Events + Features + Mission News from NASA," Newsletter, May 13, 2013, URL: http://aquarius.nasa.gov/news-status.html
30) Information provided by Sandra Torrusio, PI of the SAC-D and SAC-C missions at CONAE, Argentina
31) "NASA's Ocean Salinity Pathfinder Celebrates its First Year in Orbit," NASA, June 11, 2012, URL: http://www.nasa.gov/mission_pages/aquarius/news/first-year.html
32) Annette deCharon, "Aquarius: One Year After Launch," The Earth Observer, July - August 2012, Volume 24, Issue 4, pp: 4-9, URL: http://eospso.gsfc.nasa.gov/sites/default/files/eo_pdfs/July_Aug_2012_col_508.pdf
33) http://earthobservatory.nasa.gov/IOTD/view.php?id=78250
34) Information provided by Sandra Torrusio of CONAE (and PI of SAC-D), Buenos Aires, Argentina
35) Gary Lagerloef, "Aquarius satellite commissioning updates," January 4, 2012, URL: https://web.archive.org/web/20160310043427/http://www.esr.org/aquarius_sat/garys_blog/garys_blog.html
36) http://aquarius.nasa.gov/images/gallery/monthlysss/aq_global_dec2011.png
37) http://aquarius.nasa.gov/gallery-science.html
38) 'NASA's "Salt of the Earth" Aquarius Reveals First Map,' NASA/JPL, Sept. 22, 2011, URL: http://photojournal.jpl.nasa.gov/catalog/PIA14786
39) Alan Buis, Steve Cole, "Aquarius Yields NASA's First Global Map of Ocean Salinity," Sept. 22, 2011, URL: http://www.nasa.gov/mission_pages/aquarius/news/aquarius20110922.html
40) http://aquarius.nasa.gov/news-status.html
41) Alan Buis, Steve Cole, "Aquarius Makes First Ocean Salt Measurements," NASA, Sept. 1, 2011, URL: http://www.nasa.gov/mission_pages/aquarius/news/aquarius20110901.html
42) http://aquarius.nasa.gov/news-status.html
43) Amit Sen, Daniel Caruso, Gary Lagerloef, Sandra Torrusio, David Durham, Carlos Falcon, "Aquarius/SAC-D Mission," URL: http://www.ciccba.com.ar/archivos/PaperSateliteSAC-DAquarius2008.pdf
44) D. M. Le Vine, G. S. E. Lagerloef, F. R. Colomb, S. H. Yueh, F. A. Pellerano, "Aquarius: An Instrument to Monitor Sea Surface Salinity From Space," IEEE Transactions on Geoscience and Remote Sensing,, Vol. 45, Issue 7, July 2007, pp.:2040 - 2050
45) D. M. Le Vine, G. S. E. Lagerloef, F. R. Colomb, F. A. Pellerano, S. H. Yueh, "The Aquarius SAC/D Mission and Status of the Aquarius Instrument," Microwave Radiometry and Remote Sensing of the Environment, (Microrad 2008), March 11-14, 2008, Firenze, Italy
46) https://web.archive.org/web/20170715072848/http://www.esr.org/aquarius_sat/aquarius_main.html
47) http://aquarius.nasa.gov/pdfs/instrument.pdf
48) F. A. Pellerano, J. Piepmeier, M. Triesky, K. Horgan, J. Forgione, J. Caldwell, W. J. Wilson, S. Yueh, M. Spencer, D. McWatters, A. Freedman, "The Aquarius ocean salinity mission high stability L-band radiometer," Proceedings of IGARSS 2006 and 27th Canadian Symposium on Remote Sensing, Denver CO, USA, July 31-Aug. 4, 2006
49) N Skou, "Spaceborne L-band Radiometers: Pushbroom or Synthetic Aperture?," Proceedings of IGARSS 2004, Anchorage, AK, USA, Sept. 20•24, 2004
50) S. H. Yueh, "Microwave Remote Sensing Modeling of Ocean Surface Salinity and Winds Using An Empirical Sea Surface Spectrum," Proceedings of IGARSS 2004, Anchorage, AK, USA, Sept. 20•24, 2004
51) F. A Pellerano, K. A. Horgan, W. J Wilson, A. B Tanner, "Development of a high-stability Microstrip-based L-band radiometer for ocean salinity measurements," Proceedings of IGARSS 2004, Anchorage, AK, USA, Sept. 20•24, 2004
52) A. Freedman, D. McWatters, M. Spencer, "The Aquarius Scatterometer - An Active System for Measuring Surface Roughness for Sea-Surface Brightness Temperature Correction," Proceedings of IGARSS 2006 and 27th Canadian Symposium on Remote Sensing, Denver CO, USA, July 31-Aug. 4, 2006
53) Mark Fischman, Adam Freedman, Dalia McWatters, Andrew Berkun, Craig Cheetham, Anhua Chu, Simon Lee, Gregory Neumann, Mimi Paller, Ban Paller, Ban Tieu, John Wirth, Chialin Wu, "Development and Integration of the Aquarius Scatterometer Processor/Control Electronics for Achieving High Measurement Accuracy," Proceedings of the 2009 IEEE Radar Conference, Pasadena, CA, USA, May 4-8, 2009, paper: 3193
54) M. Fischman, A. Freedman, D. McWatters, A. Berkun, C. Cheetham, A. Chu, S. Lee, G. Neumann, M. Paller, B. Tieu, J. Wirth, C. Wu, "Low-Noise Detector with RFI Mitigation Capability for the Aquarius L-Band Scatterometer," Proceedings of the 2009 IEEE Aerospace Conference, Big Sky, MT, USA, March 7-14, 2009
55) N. Reul, J. Tenerelli, B. Chapron, D. Vandemark, "Reanalysis of Skylab S-194 L-band data in view of validating sea surface roughness corrections for salinity measurements from space," Proceedings of IGARSS 2004, Seoul. Korea, July 25-29, 2005
56) H. Marraco, L. N. Phong, "NIRST, a satellite based IR instrument for fire and sea surface temperature measurement," Proceedings of the SPIE, 'Non-Intrusive Inspection Technologies,'. Edited by Vourvopoulos, George; Doty, F. Patrick Vol. 6213, 21006,, pp. 62130J, URL: http://www.fcaglp.unlp.edu.ar/~hmarraco/trabajos/NIRST.pdf
57) François Châteauneuf, Linda Marchese, Patrice Coté, Mélanie Leclerc, Claude Chevalier, Hugo Marraco, Linh Ngo Phong, "Test Campaign Results for the Engineering Model of the SAC-D/NIRST Radiometer," Proceedings of the 7th ICSO (International Conference on Space Optics) 2008, Toulouse, France, Oct. 14-17, 2008, URL: http://www.ino.ca/Docs/Documents/publications/scientific/Test+campaign
+results+for+the_engineering+model+of+the+SAC-D-NIRST+radiometer.pdf
58) A. Linda Marchese, B. Abigail Ganopol, C. Mariano Creus, D. Martin Benitez, E. Héctor Raimondo, F. François Châteauneuf, G. Hugo Marraco, "On-ground Calibration of the Aquarius / SAC-D NIRST Radiometer," Proceedings of ASTRO 2010, 15th CASI (Canadian Aeronautics and Space Institute) Conference, Toronto, Canada, May 4-6, 2010
59) Ovidiu Pancrati, Francois Berthiaume, Fabien Claveau, Linh Ngo-Phong, Linda Marchese, François Châteauneuf, "On-orbit results of the NIRST multispectral imager," Proceedings of the 64th International Astronautical Congress (IAC 2013), Beijing, China, Sept. 23-27, 2013, paper: IAC-13-B1.3.11
60) Sonia Garcia Blanco, Timothy Pope, Patrice Coté, Mélanie Leclerc, Linh Ngo Phong, François Châteauneuf, "Radiometric packing of uncooled bolometric infrared focal plane arrays," Proceedings of the 7th ICSO (International Conference on Space Optics) 2008, Toulouse, France, Oct. 14-17, 2008, URL: http://www.ino.ca/Docs/Documents/publications/scientific/Radiometric+packaging
+of_uncooled+bolometric+infrared+focal+plane+arrays.pdf
61) Sandra Torrusio, Juan Cruz Gallo, José Kuba, "Microwave Radiometer (MWR) System Overview," 8th GPM (Global Precipitation Mission) International Planning Workshop & GDaWG, June 16-18, 2009, Paris, France, URL: http://gpm.ipsl.polytechnique.fr/index.php?option=com_docman&task=doc_download&gid=74
62) Gary Lagerloef, Raul Colomb, David LeVine, Simon Yueh, Frank Wentz, "The AQUARIUS/SAC-D Microwave Instruments for Measuring Ocean Salinity, The Water Cycle and other Applications," Proceedings of IGARSS 2008 (IEEE International Geoscience & Remote Sensing Symposium), Boston, MA, USA, July 6-11, 2008
63) Gary Lagerloef, Sandra Torrusio, "The Aquarius/SAC-D Mission - Ocean Salinity and Precipitation," 8th GPM (Global Precipitation Mission) International Planning Workshop & GDaWG, June 16-18, 2009, Paris, France, URL: http://gpm.ipsl.polytechnique.fr/index.php?option=com_docman&task=doc_download&gid=29
64) Sayak K. Biswas, Linwood Jones, Daniel Rocca, Juan-Cruz Gallio, "Aquarius/SAC-D Microwave Radiometer (MWR): Instrument description & brightness temperature calibration," Proceedings of IGARSS (International Geoscience and Remote Sensing Symposium), Munich, Germany, July 22-27, 2012
65) Héctor Raimondo, Felipe Madero, "Data Collection System (DCS) , Overview," 6th Aquarius/SAC-D Science Meeting, Seattle, WA, USA, July 19-21, 2010
66) Vittorio De Cosmo, Valeria Catalano, Stefano Landenna, Luciano Garramone, Loredana Bruca, "ROSA on Aquarius/SAC-D," SAC-D CDR (Critical Design Review), Buenos Aires, Argentina, July 21-24, 2008, URL: http://www.conae.gov.ar/satelites/ROSA.pdf
67) R. Notarpietro, G. Perona, M. Garbella, J. Wickert, "The GPS limb sounding technique for the remote sensing of atmospheric refractivity profile and the new Italian Space Agency GPS ROSA receiver," 3rd Workshop AIT on microwave remote sensing: research activities and applications, Napoli, Italy, 2004
68) A. Zin, S. Zago, E. Mangolini, S. Landenna, L. Marradi, S. Radicella, B. Nava, R. Notarpietro, V. Catalano, "The ROSA Radio Occultation Project," Proceedings of NAVITEC 2012, 6th ESA Workshop on Satellite Navigation Technologies, ESA/ESTEC, Noordwijk, The Netherlands,Dec. 5-7, 2012
69) R. Ecoffet, S. Barde, J. Cueto, D. Falguere, T. Nuns, S. Duzellier, D. Boscher, S. Bourdarie, R. Colomb, C. Alomzo, C. Hoffman, "Observations of the dynamics of the radiative environment in polar orbit in the period 11/2000-11/2001," URL: http://www.esa-spaceweather.net/spweather/workshops
/SPW_W3/PROCEEDINGS_W3/SWWorkshop_Ecoffet2.PDF
70) "Satélite de Aplicaciones Cientificas-D (SAC-D) Network Requirements Document," NASA/GSFC, 450-NRD-SAC-D, Revision 1, Sept. 14, 2010, URL: http://oceancolor.gsfc.nasa.gov/AQUARIUS/DOCS/450-NRD-SAC-D-R1.pdf
The information compiled and edited in this article was provided by Herbert J. Kramer from his documentation of: "Observation of the Earth and Its Environment: Survey of Missions and Sensors" (Springer Verlag) as well as many other sources after the publication of the 4th edition in 2002. - Comments and corrections to this article are always welcome for further updates (eoportal@symbios.space).
Overview Spacecraft Launch Mission Status Sensor Complement Ground Segment References Back to top