Lunar IceCube
Non-EO
NASA
Quick facts
Overview
Mission type | Non-EO |
Agency | NASA |
Launch date | 16 Nov 2022 |
Lunar IceCube
Spacecraft Launch Secondary Payloads Sensor Complement References
Lunar IceCube is a selected NASA nanosatellite mission to prospect, locate, and estimate size and composition of water ice deposits on the Moon for future exploitation by robots or humans.
Lunar IceCube is one of several public-private partnerships chosen under NASA’s NextSTEP (Next Space Technologies for Exploration Partnerships) Broad Agency Announcement for the development of advanced exploration systems. Among the first small satellites to explore deep space, Lunar IceCube will help lay a foundation for future small-scale planetary missions, mission scientists said. In addition to providing useful scientific data, Lunar IceCube will help inform NASA’s strategy for sending humans farther into the solar system. The ability to search for useful assets can potentially enable astronauts to manufacture fuel and other provisions needed to sustain a crew for a journey to Mars, reducing the amount of fuel and weight that NASA would need to transport from Earth. 1) 2) 3) 4)
MSU (Morehead State University in Kentucky) is leading the 6U CubeSat mission, with significant involvement from scientists and engineers at NASA/GSFC (Goddard Space Flight Center) in Greenbelt, Maryland, and the Massachusetts-based Busek Company.
Under the university-led partnership, MSU's Space Science Center will build the 6U nanosatellite and provide communications and tracking support via its 21 m ground station antenna. Busek will provide the state-of-the-art electric propulsion system and Goddard will construct IceCube’s only miniaturized instrument, the Broadband InfraRed Compact High Resolution Explorer Spectrometer (BIRCHES). The instrument will prospect for water in ice, liquid, and vapor forms from a highly inclined elliptical lunar orbit. Goddard also will model a low-thrust trajectory taking the pint-size satellite to lunar orbit with very little propellant.
“Goddard scientists and engineers have deep experience in areas that are critical to interplanetary exploration,” said mission Morehead State University Principal Investigator Benjamin Malphrus, explaining why the university teamed with Goddard. “The significant expertise at Goddard, combined with Morehead State’s experience in smallsats and Busek’s in innovative electric-propulsion systems, create a strong team.”
The Lunar IceCube mission science focus, led by the JPL Science PI (Pamela E. Clark), is on:
1) enabling broadband spectral determination of composition and distribution of volatiles in regoliths of the Moon and analogous bodies as a function of time of day, latitude, regolith age and composition;
2) providing geological context by way of spectral determination of major minerals; and
3) thus enabling understanding of current dynamics of volatile sources, sinks, and processes, with implications for evolutionary origin of volatiles. The mission is designed to address NASA HEOMD (Human Exploration and Operations Mission Directorate) Strategic Knowledge Gaps related to lunar volatile distribution (abundance, location, and transportation physics of water ice).
Lunar IceCube amplifies and extends the findings of previous missions. While the M3 (Moon Mineralogy Mapper) on the Chandrayaan-1 mission of ISRO provided a ‘snapshot’ mosaic of the lunar nearside, indicating surface coating of OH/H2O near the poles, Lunar Ice Cube will provide coverage of the same swaths as a function of latitude at several times of day. Lunar Ice Cube will extend evidence for diurnal variation in OH absorption provided by Deep Impact and other C-, H-, O-, and S-bearing volatiles provided by LCROSS (Lunar CRater Observation and Sensing Satellite) through geospatial linkage. The Lunar Ice Cube measurements will completely encompass the broad 3 µm band resulting from absorption by several forms of water instead of cutting off at 3 µm as previous NIR spectrometers have done.
Mission | Finding | IceCube |
Cassini VIMS, Deep Impact | Surface water detection, variable hydration, with noonpeak absorption | Water & other volatiles, fully characterize |
Chandrayaan M3 | H2O and OH (<3 µm) in mineralogical context nearside snapshot at one lunation | |
LCROSS | Ice, other volatile presence and profile from impact in polar crater | |
LP, LRO, LEND, LAMP, | H+ in first meter (LP, LEND) & at surface (LAMP) inferred as ice |
The primary science goal of the Lunar IceCube mission is to investigate the distribution of water as a function of time of day, latitude, and regolith composition in the context of lunar mineralogy. Lunar IceCube will extend the findings of previous missions. 5) While Chandrayaan M3 provided a ‘snapshot’ mosaic of the lunar nearside, indicating surface coating of OH/H2O near the poles, Lunar IceCube will provide coverage of the same swaths as a function of latitude at several times of day. Lunar Ice Cube will extend evidence for diurnal variation in OH absorption provided by Deep Impact and other C-, H-, O-, and S-bearing volatiles provided by LCROSS through geospatial linkage. Lunar IceCube measurements will completely encompass the broad 3 µm band resulting from absorption by water in the solid and vapor states. 6)
Lunar IceCube will include the Broadband InfraRed Compact High Resolution Exploration Spectrometer (BIRCHES), developed by GSFC- a compact version of the successful New Horizons instrument designed with the high spectral resolution (5 nm) and wavelength range (1 to 4 µm) needed to distinguish forms of water, including ice. The mission will complement the scientific work of other missions by focusing on the abundance, location and transportation physics of water ice on the lunar surface at a variety of latitudes. Working together as the first “ad hoc” constellation of interplanetary CubeSats exploring the Moon, Lunar IceCube, Lunar Flashlight and LunaH-Map have the potential to lend significant insight into the location, depth, cyclic nature and transport mechanisms of water on the Moon. 7)
Lunar IceCube, while primarily a science mission, will demonstrate technologies that will enable future interplanetary exploration with small satellite platforms including radiation-hardened subsystems, a precise ranging transponder/transceiver, a capable attitude determination and control system, a high power solar array and an innovative electric propulsion system (EP). The EP (Busek BIT-3 Iodine engine) generates 1.2 km/s-1 of ΔV and, combined with an innovative low energy manifold trajectory, allows the spacecraft to reach lunar orbit from Earth escape with minimal energy. The 13 secondary payloads to be deployed on Artemis 1, including Lunar IceCube, will usher in a new era of solar system exploration with small satellite platforms.
Spacecraft
Development of the Lunar IceCube deep space CubeSat bus leverages MSU’s CubeSat mission experience, utilizes systems with significant flight heritage (in LEO), and incorporates new NanoSat technologies to develop an evolved, radiation-tolerant 6U CubeSat that can support interplanetary investigator science. The 6U Lunar IceCube is based on MSU’s bus heritage and incorporates high power generation (120 W of continuous power in cislunar space adapted by Pumpkin Inc. from their lower power Supernova design), a radiation-hardened flight computer (Proton 400K made by Space Micro, Inc.) , a highly-capable micronized GNC (Guidance, Navigation and Control) system and BCT's (Blue Canyon Technologies) XACT attitude determination and control subsystem. Several options were considered for communications including COTS systems and a high throughput X-band communication system designed by JPL for lunar CubeSat missions. The JPL X-band radio, known as Iris, was selected. Iris 2.1 is a full transponder that is capable of high data throughput and has Doppler ranging capabilities.
The 6U Lunar IceCube bus was essentially derived from MSU’s successful 2U CXBN (Cosmic X-ray Background NanoSatellite) mission and 1U KySat-2 missions. Additionally, several of the subsystems have successfully flown on numerous NanoSat and MicroSat missions and most of the COTS subsystems will have flight heritage prior to the development of a complete Lunar IceCube flight unit.
The spacecraft design is at a CDR level of maturity; an up-to-date specification overview is provided in Table 1, with Figure 2 and Figure 3 showing the subsystem layout. The combination of flight qualified hardware and innovative solutions to difficult engineering challenges provides for a robust spacecraft bus solution for the Lunar IceCube program.
ADCS (Attitude Determination and Control Subsystem): Attitude control will be provided by the BCT( Blue Canyon Technology) XACT which is an integrated ADCS. This fully integrated system includes star trackers, IMU, and RWAs flight heritage on the MinXSS mission, and can interface with thrusters. Several of the NASA EM-1 CubeSats utilize the BCT XACT.
C&DH (Command and Data Handling): The C&DH selected for Lunar IceCube is the Space Micro Inc. Proton P400K-SGMII-2-PCI104S-SD Space Computer. The Proton400K TM computing platform is a high performance, low power radiation hardened processing solution that meets the challenge of the space environments. This product utilizes the Freescale advanced 45 nm dual-core microprocessor and combined with Space Micro's patent pending radiation mitigation technologies.
RF communications: Communications with the Lunar IceCube are provided by the X-band JPL Iris Radio and dual patch antennas. MSU has a 21 m dish antenna that is becoming part of the DSN. Anticipated data rate~128 kbit/s with the 34 m DSN antennas and 64 kbit/s with the MSU 21 m Ground Station. — To ensure commonality in spacecraft communications and to reduce costs, a CubeSat compatible deep space transponder developed by JPL will be used by several of the EM-1 secondary payloads (Figure 1). The Iris V2 X-band radio is also adaptable for Ka-band, S-band, and UHF (Ultra High Frequency) options. The Iris radio weighs approximately 0.875 kg, and it has an output power of 4 W (Ref. 8). 8)The Iris radios and the MSU ground station will incorporate Disruption Tolerant Networking technology to enable more efficient store and forward communications protocols (Ref. 17).
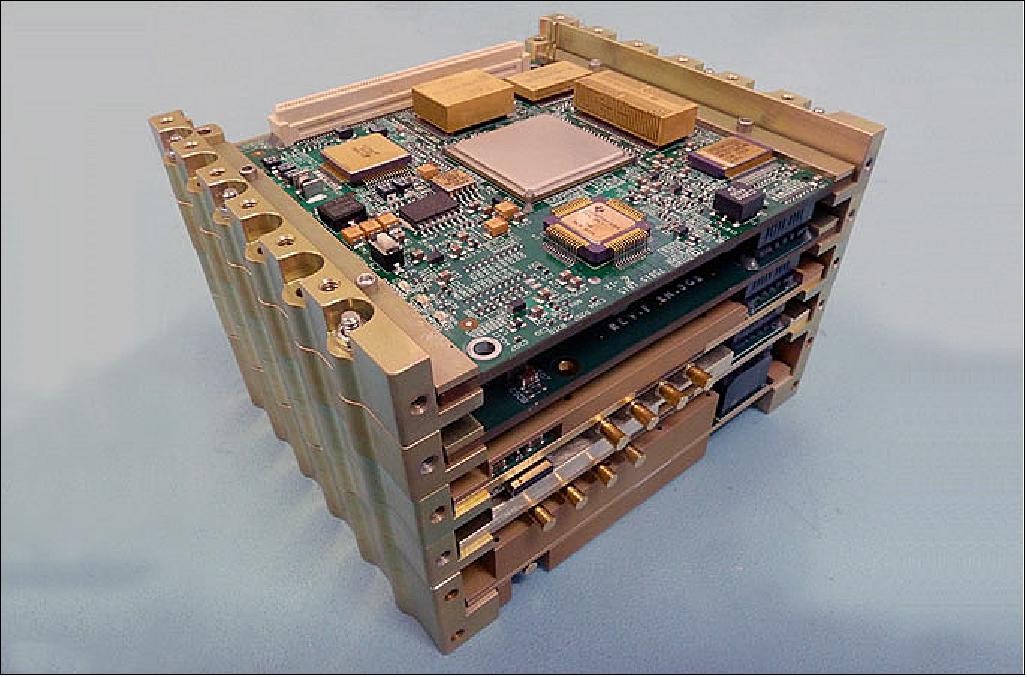
Launch mass | ~14 kg |
Payload mass capability, volume | 3.5 kg, 2.0 U |
ADCS pointing accuracy | ±.14 arcsec (1σ) |
Orbit knowledge | 10 m, 0.15 m/s |
Maneuver rate | 3º/s |
Payload power capability | 17.8 W |
Prime power generated | 120 W continuous |
Voltages available | 28 V, 12 V, 5 V, 3.3 V |
Performance of BIT-3 RF Ion Propulsion System | Nominal thrust: 1.24 mN |
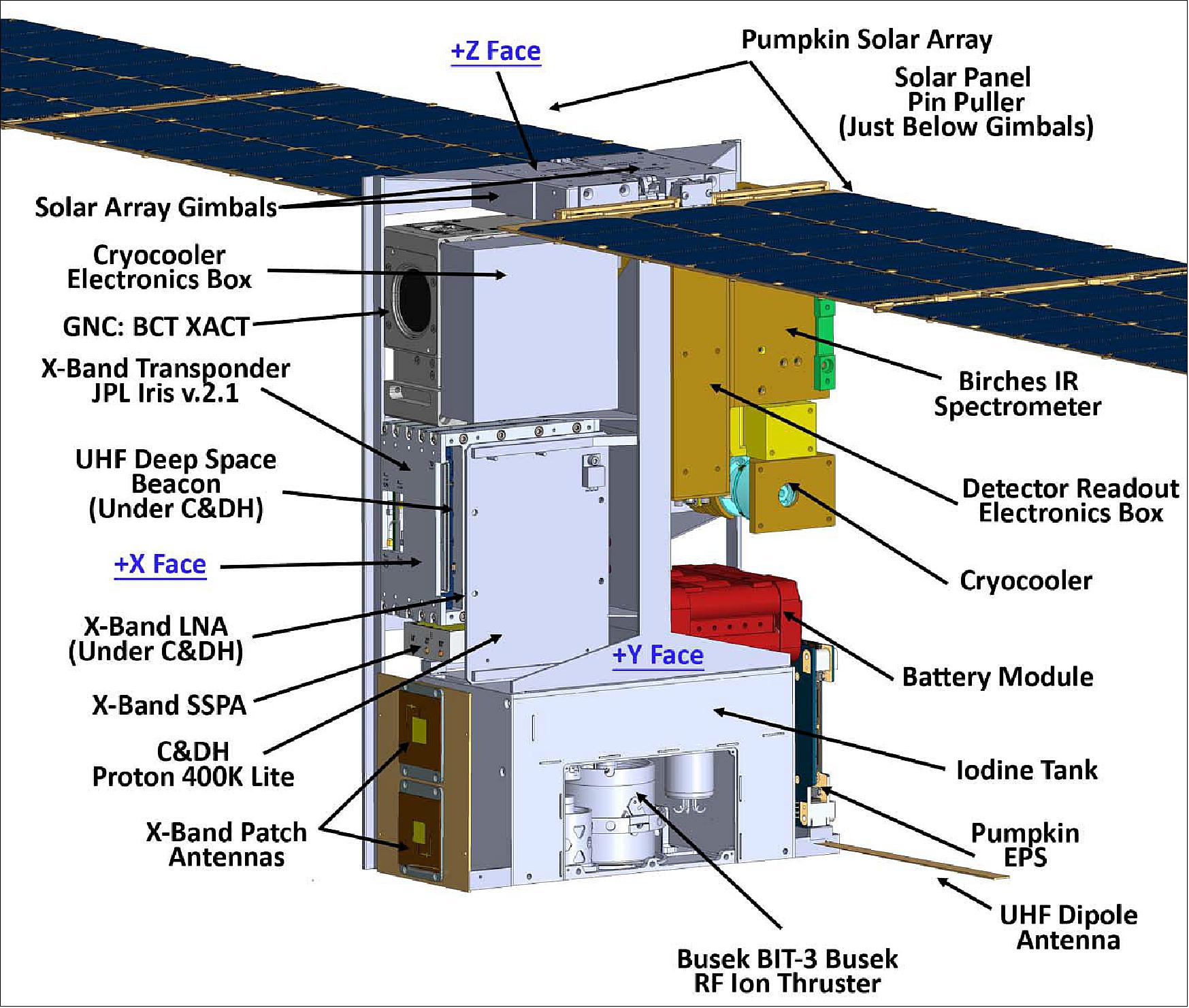
Propulsion: The Busek Company of Natik, MA, with sponsorship from NASA, developed a low-thrust electric propulsion system named BIT-3 (Busek Ion Thruster-3 cm grid). The BIT-3 system is capable of delivering variable Isp and thrust of 2,250 s and 1.24 mN, respectively, at the designed power of 70 W. The BIT-3 RF ion thruster is regarded as the world’s first gridded ion thruster ever to operate on iodine propellant. 9) 10)
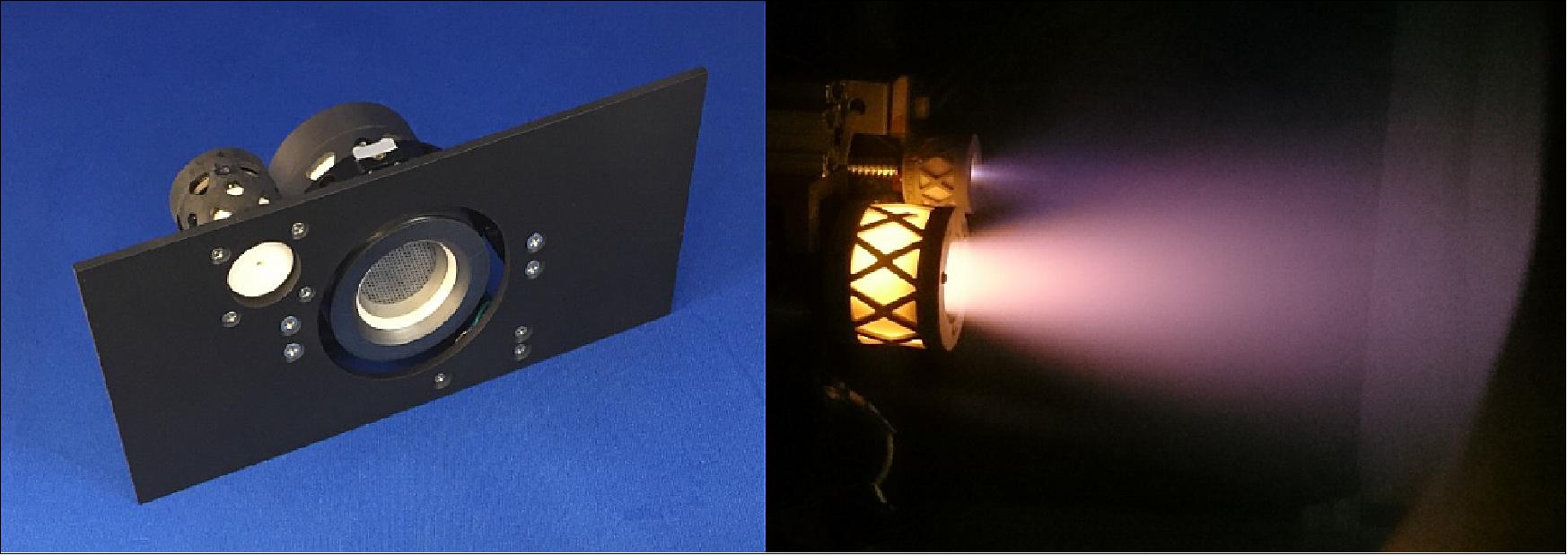
The concept of utilizing iodine (I2) as an EP propellant was identified over a decade ago and patented by the U.S. Air Force. Busek pioneered the practical application of such propellant in 2010 with the development of an iodine-fueled 200 W HET (Hall Effect Thruster) known as BHT-200. This development has led to a patent involving the iodine feed system that was the departure point of all Busek’s iodine EP technologies. Since then, Busek has successfully demonstrated iodine-fueled HETs up to 10 kW power and verified that I2 has very similar performance characteristics compared to the legacy EP propellant xenon (Xe).
I2 has many advantages over Xe from the perspective of storage and handling requirement, launch safety, and cost. Table 1 compares the physical properties between these two propellants. I2 appears naturally as solid crystal in diatomic form, with relatively-low dissociation energy at around 1.54 eV. Atomic iodine (I) is only slightly lighter than Xe, and its electron impact ionization cross section is larger and first ionization potential is lower. These characteristics make I2-based plasma just as easy to create and efficient to use for propulsion purpose as with xenon. Scientists used to have reservation regarding I2’s dissociation energy cost may reduce an EP device’s overall thrust efficiency; this turned out to be a non-issue as reported by previous HET test results. It is true that in a plasma generator some of the I2 molecules may become ionic dimers (I2+) without dissociating, but as James Szabo and Mike Robin point out, these species are relatively low in percentage (<10%) and most of the propellant exit as singly-charged monomer I+. 11)
Figure 3 shows a conceptual flow diagram of an iodine BIT-3 propulsion unit, with both the thruster and the neutralizer operating on a single iodine storage and feed system. During operation, the propellant reservoir is heated to produce iodine vapor via sublimation, which is then fed to the BIT-3 thruster and the BRFC-1 neutralizer (an iodine-compatible RF cathode). The feed lines and flow control valves are heated to above the tank temperature in order to prevent condensation. The vapor flow rate is controlled by the tank heater, with feedback from the tank’s temperature sensors (primary), measured ion beam current (secondary), and pressure transducer (tertiary backup). The control scheme works best for long-duration, steady-state maneuvers as the response time between applied heater power and actual I2 flow rate change can be slow.
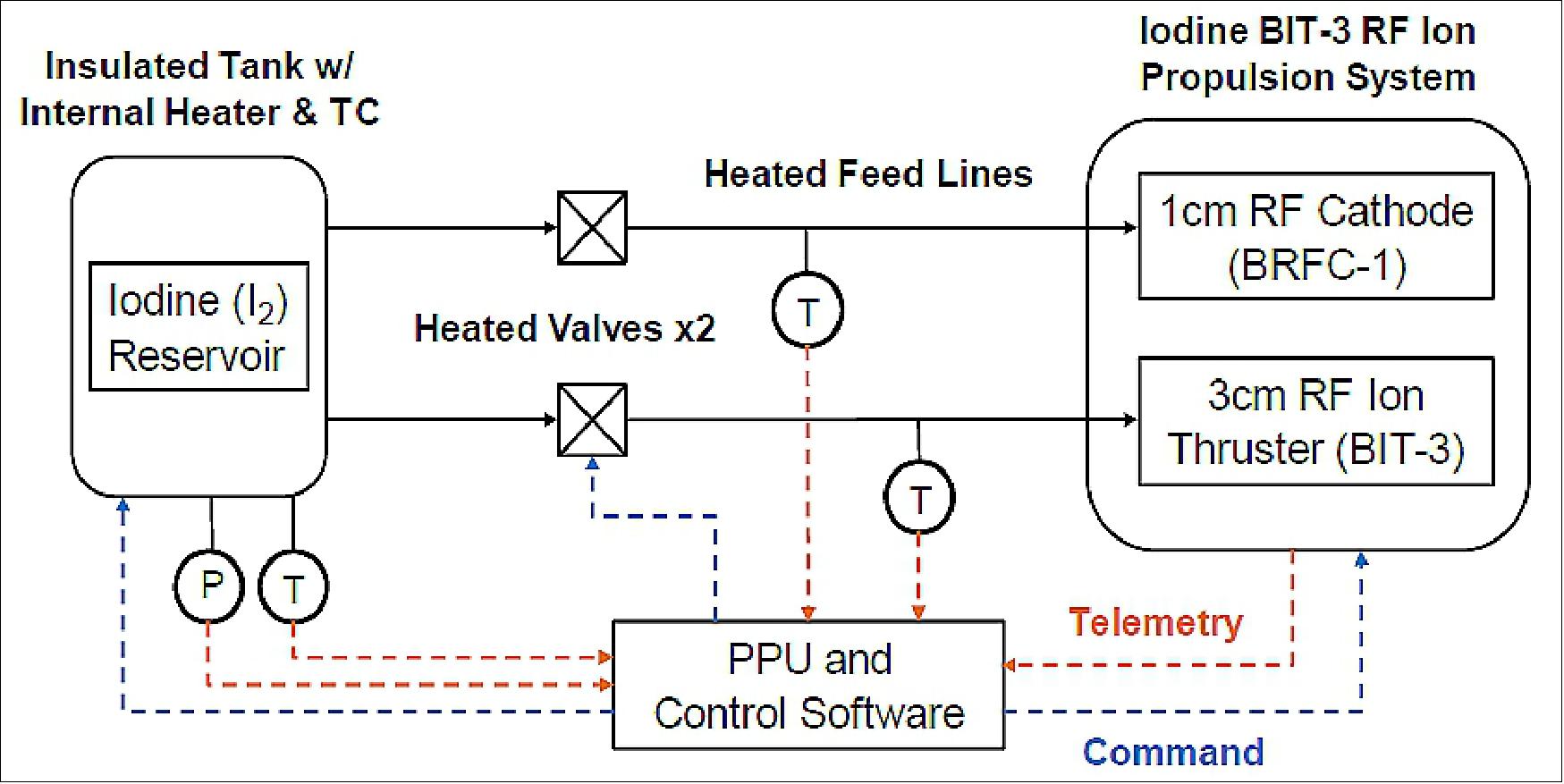
Figure 4 shows the configuration of the BIT-3 flight system currently in development. The system envelope is 180 x 88 x 102 mm (~1.6U) with a wet mass of 3 kg. In addition to the thruster and the neutralizer, other notable subsystem components include a 1.5 kg-capacity iodine storage tank, a custom 2-axis gimbal integrated to the thruster body, a highly miniaturized and radiation-tolerant PPU (Power Processing Unit), and COTS (Commercial-of-the-Shelves) pressure transducer and iodine-compatible control valves. The 2-axis gimbal utilizes flight-grade stepper motors and is capable of ±10º actuation and 0.1º accuracy. The gimbal enables the single primary thruster to de-saturate the RWA (Reaction Wheel Assembly) while in deep space. It is also capable to balance out any C.G. shift in-flight as the propellant is consumed.
The expected performance of the iodine BIT-3 system is currently based on actual thrust and Isp data obtained with I2 propellant, with some estimation on the heater power consumption and FM PPU efficiency. The flight BIT-3 system will be throttleable between 56 and 80 W at the PPU input and capable of accepting 28-37 V unregulated voltage and RS-485 command. Expected thrust and Isp within this power range are 0.66-1.24 mN and 1,400-2,640 s, respectively. The 80 W max power is not outrageous for 6U CubeSats, as current SOA (State-of-the-Art) solar array for 6U can generate up to 120 W prime power at 1 AU distance from the Sun. When given sufficient power, the iodine BIT-3 system can provide a 6U/14 kg CubeSat an unprecedented ΔV capability of 2.9 km/s. The BIT-3 system has also been recognized as having the highest volumetric impulse (total impulse per unit volume) among all SOA CubeSat propulsion options.
In summary, the iodine BIT-3 propulsion system offers extremely high Isp (>2,000 s) heretofore unavailable to nanosatellites. This type of propulsion technology would enable a variety of deep space CubeSat missions including lunar reconnaissance, asteroid rendezvous, inner planet flyby, as well as low flying Earth observation.
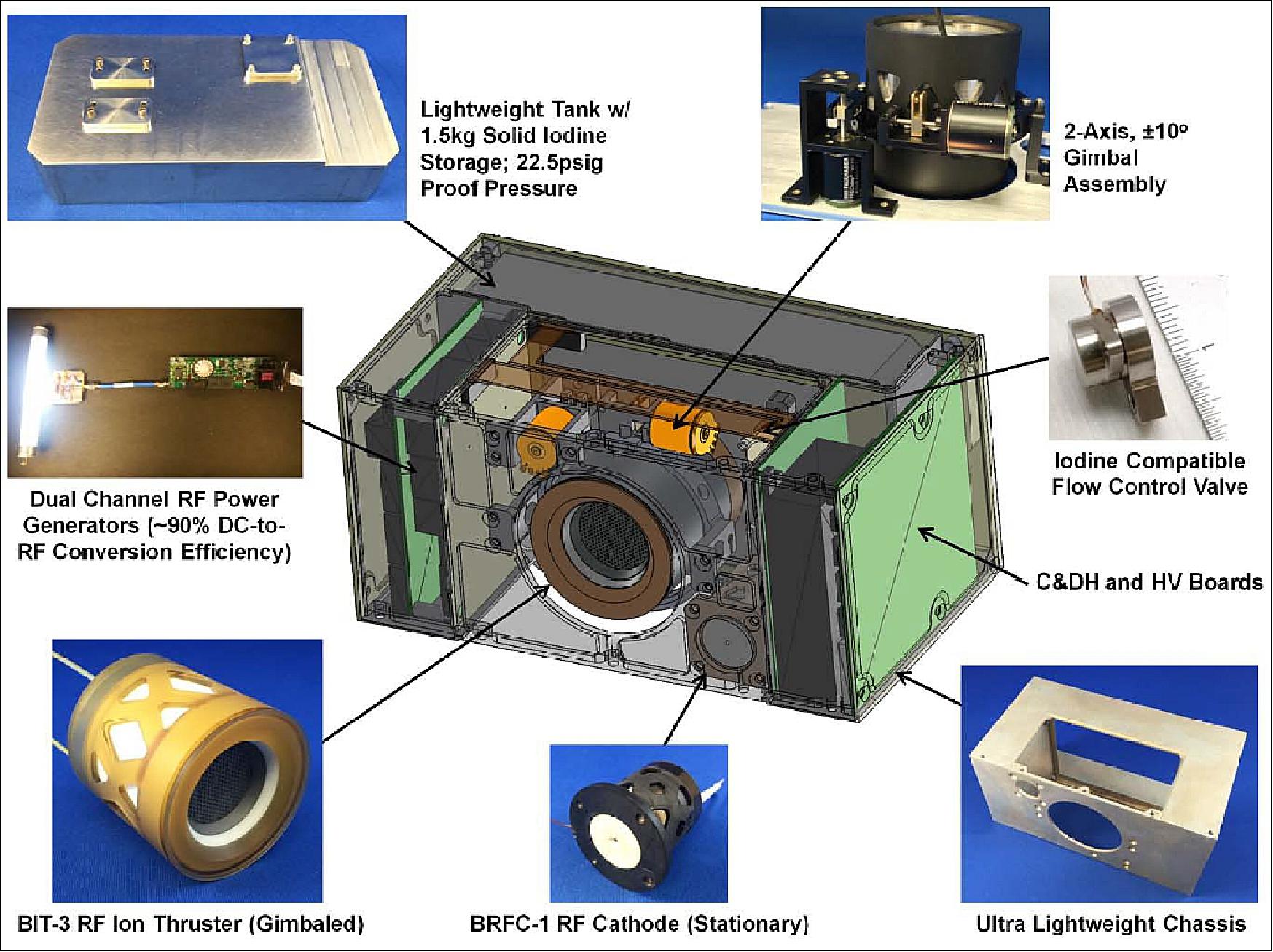
The 6U spacecraft (Figure 2) utilizes and demonstrates the functionality for deep space of the Busek High ISP RF Ion Engine for propulsion, the BCT attitude knowledge and control system (XACT Star Tracker, Reaction Wheels, IMU), version 2.1 of the JPL Iris ranging transceiver, and the inexpensive Space Micro flight computer, designed to be radiation tolerant.
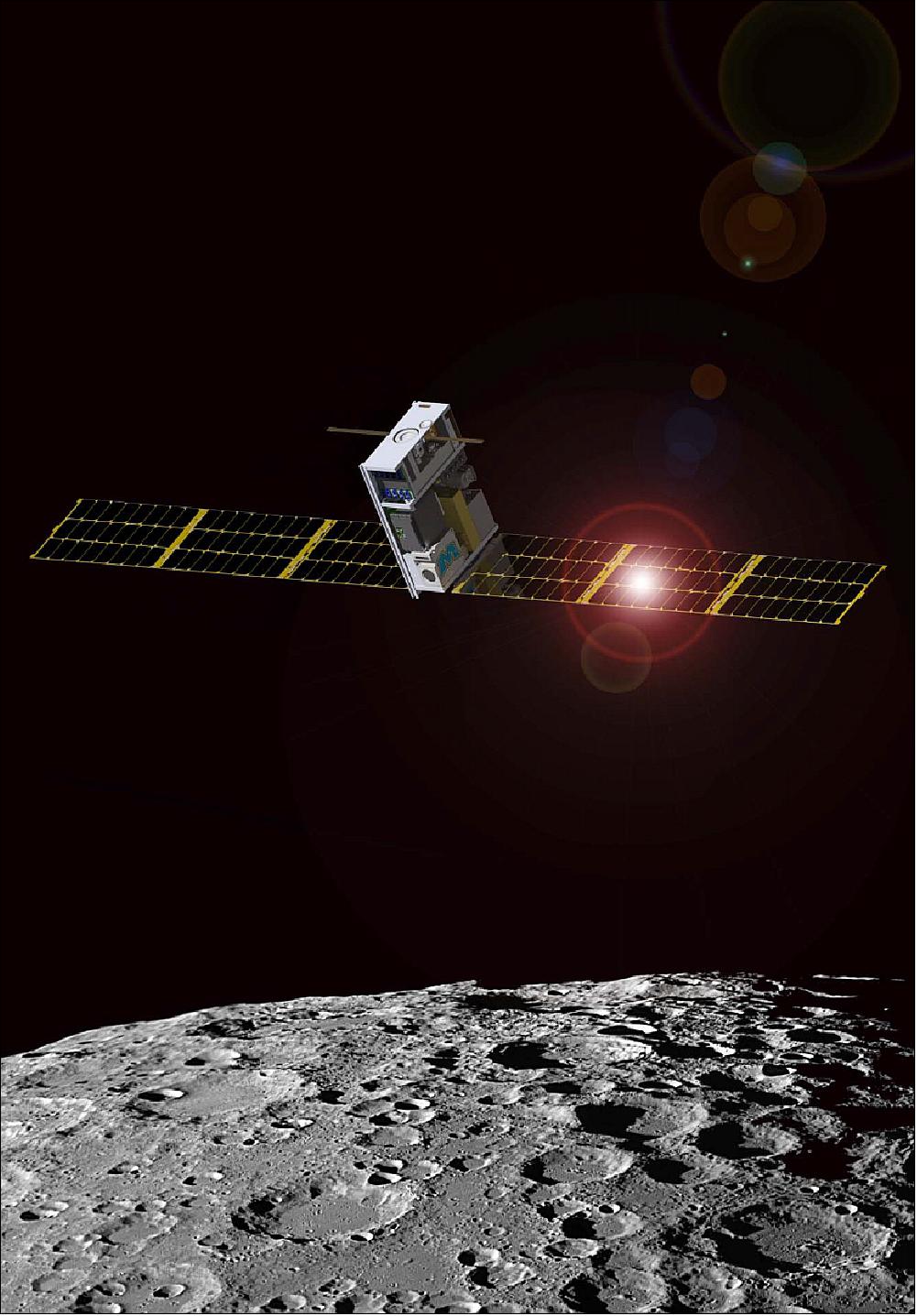
Development status of the Lunar IceCube
• June 8, 2021: The Lunar IceCube CubeSat successfully passed essential environmental testing at NASA’s Goddard Space Flight Center in Greenbelt, Maryland. The spacecraft, pictured above, will fly aboard the upcoming Artemis I mission to the Moon as a secondary payload to investigate the amount and distribution of water ice on the Moon. 12)
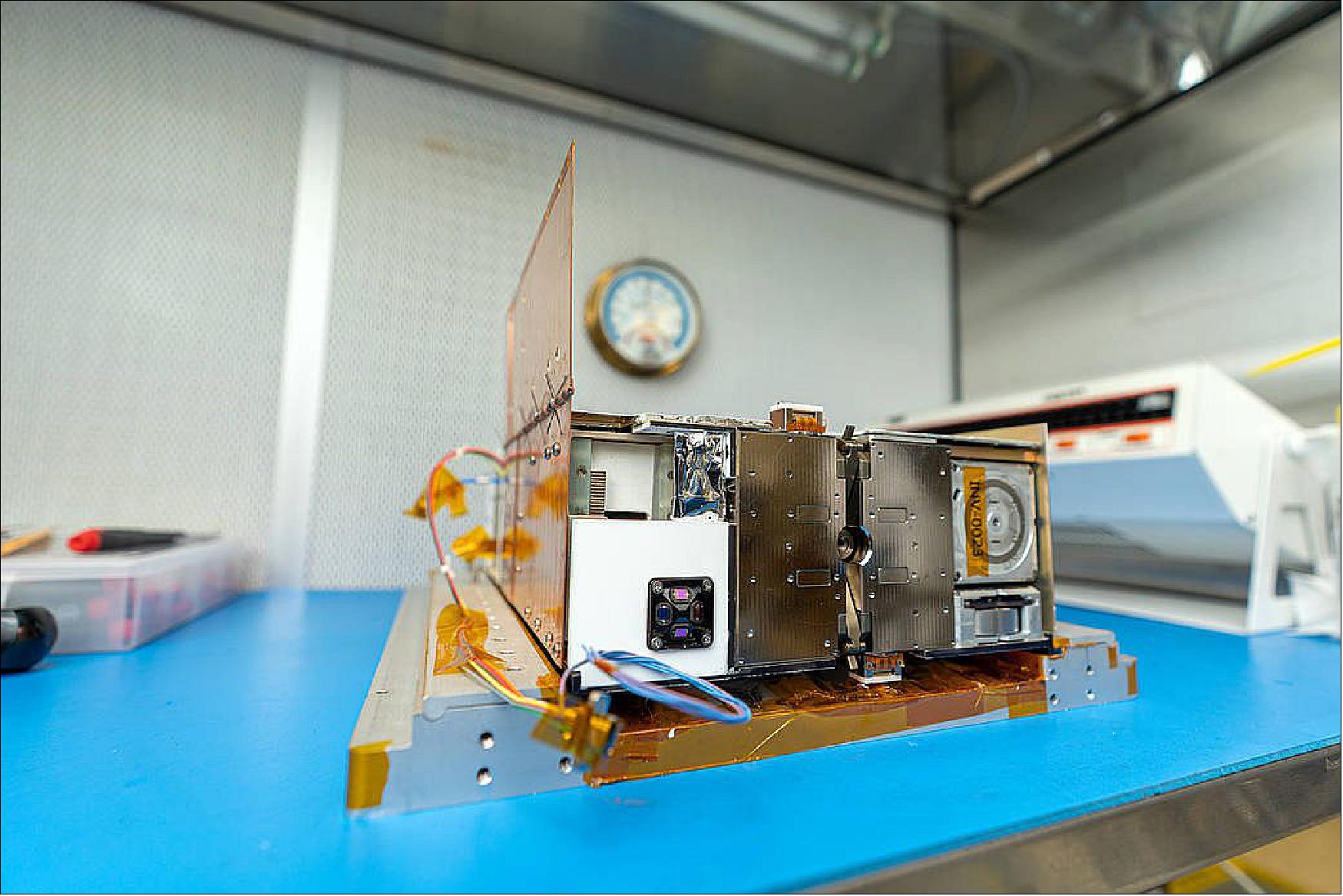
- The spacecraft must go through intensive testing on Earth before flight to ensure the systems can survive a rocket launch and perform in the harsh environment of space. Lunar IceCube’s testing included thermal vacuum, which replicated the vacuum of space by draining the atmosphere from the chamber and exposing the spacecraft to extreme hot and cold temperatures.
- Once it reaches orbit, Lunar IceCube will begin its mission. NASA’s Artemis program is returning humanity to the Moon, but this time to stay. By understanding the dynamics of water and other substances on the Moon that easily turn to vapor or gas, scientists will be able to predict seasonal changes and determine possible uses for lunar resources such as water.
- Engineers also conducted instrument testing and calibration for the Broadband InfraRed Compact High-Resolution Exploration Spectrometer (BIRCHES) on Lunar IceCube that will help map the distribution and dynamics of water on the Moon’s surface. This helped verify the instrument’s functionality.
- Lunar IceCube is funded by NASA’s Next Space Technologies for Exploration Partnerships program in support of NASA’s Advanced Exploration Systems Division within the Human Exploration and Operations Mission Directorate. The Lunar IceCube mission is led by Morehead State University in Morehead, Kentucky, NASA’s Goddard Space Flight Center, NASA’s Jet Propulsion Laboratory in Southern California, NASA’s Katherine Johnson Independent Verification and Validation Facility in Fairmont, West Virginia, and Busek Space Propulsion and Systems in Natick, Massachusetts.
Launch
Lunar IceCube is a selected NASA nanosatellite mission to prospect, locate, and estimate size and composition of water ice deposits on the Moon for future exploitation by robots or humans. It will fly as a secondary payload mission on the first flight of the SLS (Space Launch System), EM-1 (Exploration Mission-1) expected to launch in 2021. 13)
The satellites selected for EM-1 will be installed inside the adapter, which connects Orion to the upper stage of NASA’s newest rocket — the SLS launch vehicle designed to ferry humans and gear around the moon and beyond. Once the rocket reaches a certain position on its way to the moon, ground controllers will send a command to release the payloads, which will follow their own trajectories to their final destinations in and around the moon.
Busek’s RF Ion BIT-3 thruster, along with a carefully designed trajectory modeled by Goddard’s state-of-the-art trajectory-design software, will get IceCube to its destination in about three months, according to Dave Folta, the Goddard orbital engineer who has developed advanced tools for modeling lunar orbits for spacecraft equipped with both chemical and low-thrust propulsion systems.
The journey of the Lunar IceCube will begin after deployment— and will be another challenge given the miniscule real estate set aside for propellant. Ground controllers will fire Busek’s miniaturized electric thrusters — the world’s only propulsion system powered with an iodine propellant — driving the spacecraft along a complex path that uses the gravity of the sun, Earth and moon, looping around Earth a couple times and then to its destination. Because the thrusters operate electrically using small amounts of propellant, an orbital path that takes advantage of gravitational acceleration from the Earth and moon is vital. This approach utilizes the interplanetary superhighway- a low energy trajectory using gravitational manifolds.
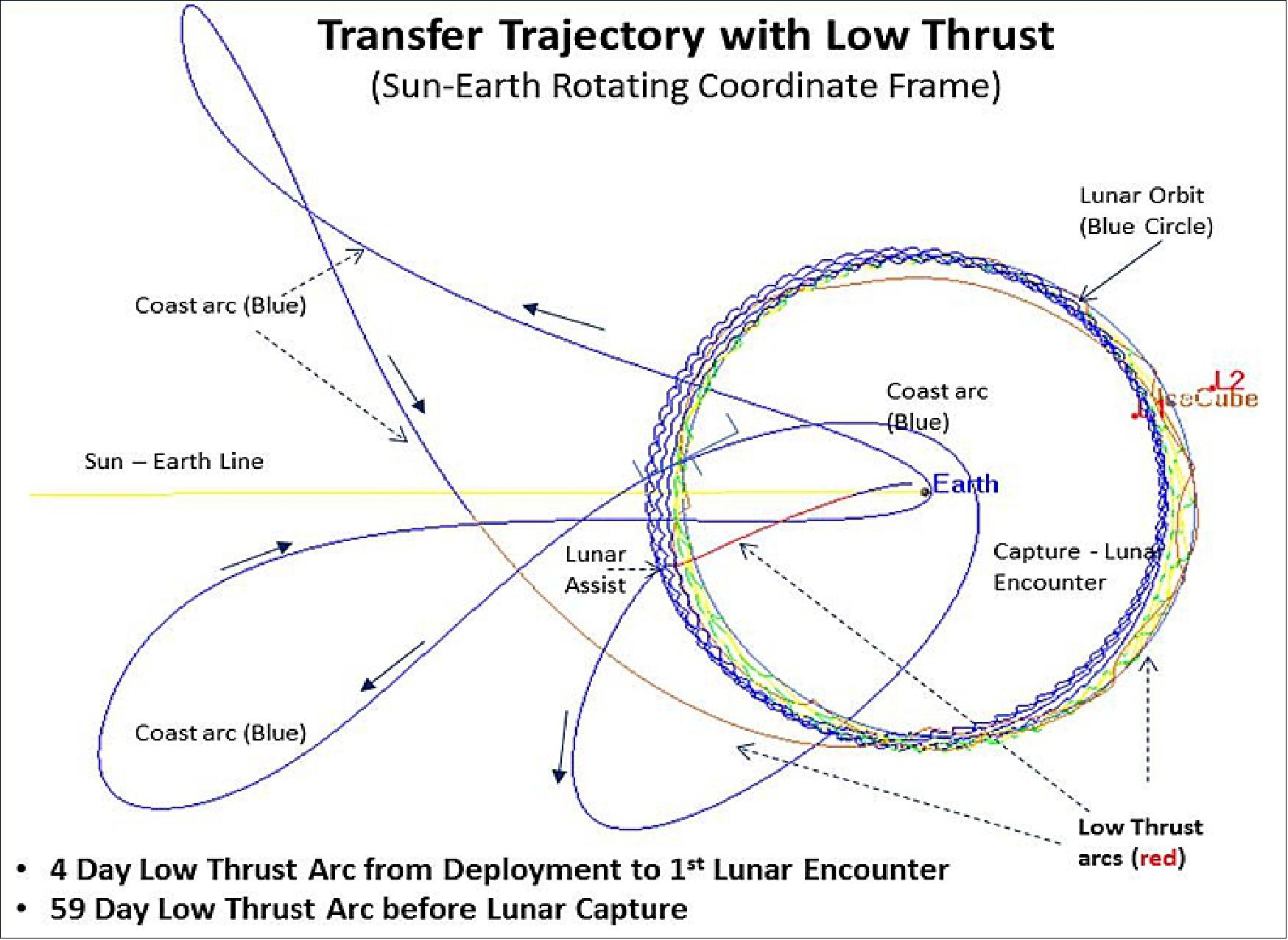
Lunar IceCube will fly in a nearly polar, highly elliptical, equatorial periapsis orbit designed to provide repeat coverage of the same orbital swaths systematically at varying times of day during up to six consecutive lunar cycles (Figure 9). In this way, such overlapping coverage will be provided for up to 10% of the lunar surface across all latitudes. 14)
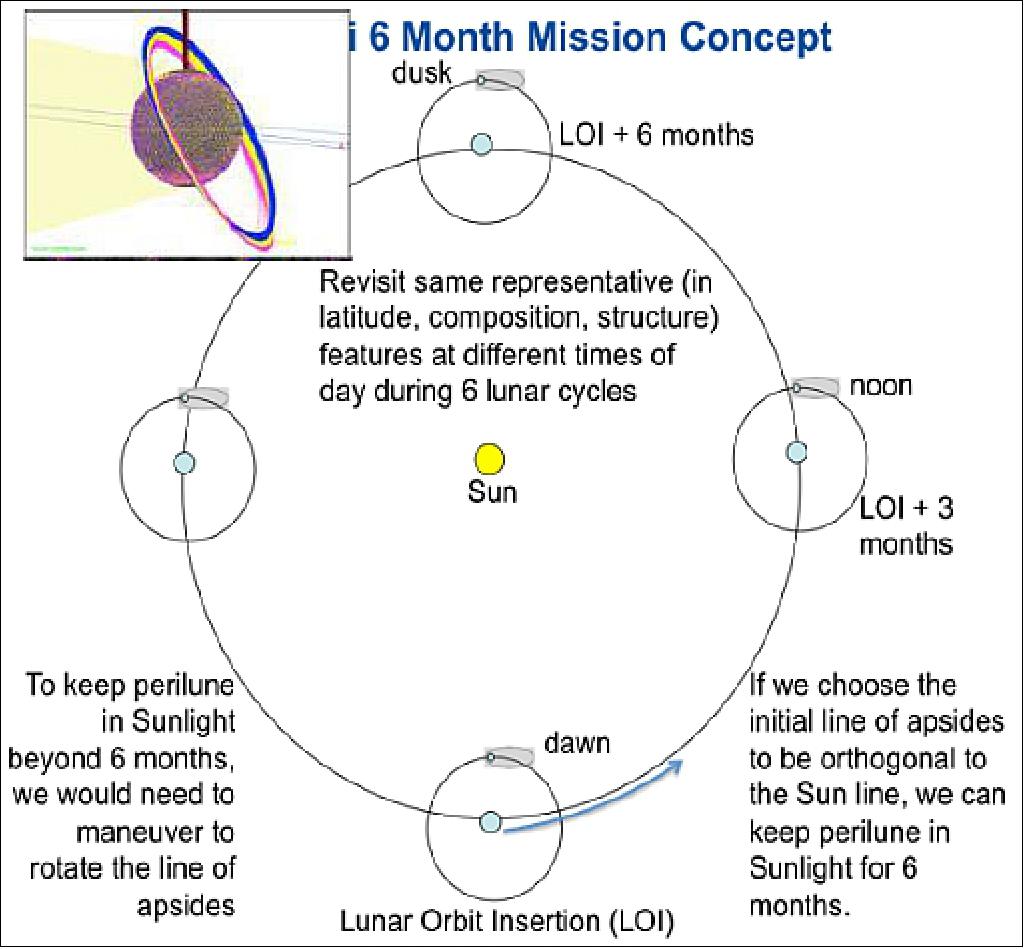
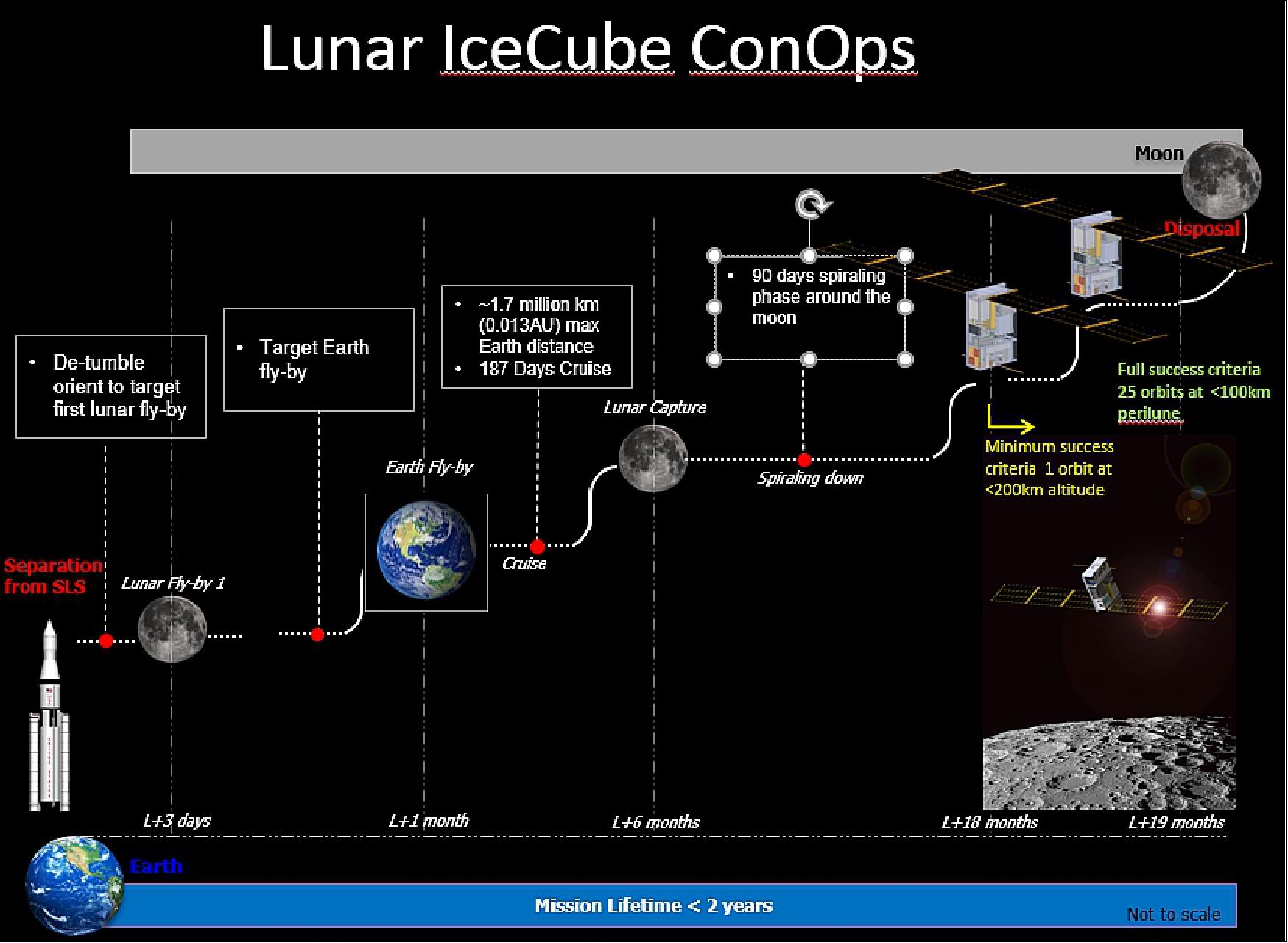
Secondary payloads of Artemis-1 (formerly Orion/EM-1)
The first flight of NASA’s new rocket, SLS ( Space Launch System), will carry 13 CubeSats/Nanosatellites to test innovative ideas along with an uncrewed Orion spacecraft in 2020. These small satellite secondary payloads will carry science and technology investigations to help pave the way for future human exploration in deep space, including the journey to Mars. SLS’ first flight, referred to as EM-1 (Exploration Mission-1 ), provides the rare opportunity for these small experiments to reach deep space destinations, as most launch opportunities for CubeSats are limited to low-Earth orbit. 16) 17)
The secondary payloads, 13 CubeSats, were selected through a series of announcements of flight opportunities, a NASA challenge and negotiations with NASA’s international partners.
Overview of the 10 selected US secondary missions for the inaugural Orion/EM-1 test flight plus three CubeSats from international partners 18) 19)
NASA selected two payloads through the NextSTEP (Next Space Technologies for Exploration Partnerships) Broad Agency Announcement of May 5, 2015:
• LunIR ⟨originally called Skyfire) - Lockheed Martin Space Systems Company, Denver, Colorado, will develop a 6U CubeSat to perform a lunar flyby of the moon, taking sensor data during the flyby to enhance our knowledge of the lunar surface. LunIR will test a new technology mid-wave infrared camera and micro-cryocooler. LunIR also includes a visible imager.
• Lunar IceCube - Morehead State University, Kentucky, will build a 6U CubeSat to search for water ice and other resources at a low orbit of only 62 miles above the surface of the moon. This CubeSat will orbit the Moon and prospect for water and other volatiles in lunar regolith using BIRCHES (Broadband Infrared Compact High-Resolution Exploration Spectrometer) developed at NASA/GSFC. 18)
Three payloads were selected by NASA’s Human Exploration and Operations Mission Directorate:
• NEA Scout (Near-Earth Asteroid Scout), the 6U CubeSat of NASA/MSFC/JPL will perform reconnaissance of an asteroid, take pictures and observe its position in space
• BioSentinel - a 6U CubeSat of NASA/ARC will use yeast to detect, measure and compare the impact of deep space radiation on living organisms over long durations in deep space.
• Lunar Flashlight - a 6U CubeSat of NASA/JPL/MSFC will look for ice deposits and identify locations where resources may be extracted from the lunar surface
Two payloads were selected by NASA’s Science Mission Directorate:
• CuSP – a 6U CubeSat of the SwRI (Southwest Research Institute) “space weather station” to measure particles and magnetic fields in space, testing the practicality for a network of stations to monitor space weather
• LunaH-Map a 6U CubeSat of Arizona State University will map hydrogen within craters and other permanently shadowed regions throughout the moon’s south pole
Three additional payloads were determined through NASA’s Cube Quest Challenge – sponsored by NASA’s Space Technology Mission Directorate and designed to foster innovations in small spacecraft propulsion and communications techniques. CubeSat builders will vie for a launch opportunity on SLS’ first flight through a competition that has four rounds, referred to as ground tournaments, leading to the selection in 2017 of the payloads to fly on the mission.
• Cislunar Explorers, Cornell University, Ithaca, New York. Cislunar Explorers’ concept consists of a pair of spacecraft on a mission to orbit the moon. These two spacecraft are mated together as a 6U CubeSat. After deployment from the launch vehicle, they will split apart and each give their initial rotation in the process of decoupling.
• CU-E3(CU Earth Escape Explorer), a 6U CubeSat of the University of Colorado in Boulder, CO. The CU-E3 mission will use a lunar gravity assist maneuver to place the CubeSat in a heliocentric orbit that trails the Earth at a distance > 1AU (Astronomical Unit). The distance between the Earth and the spacecraft will gradually increase over time, reaching 27 million km by the end of its one-year mission.
• Team Miles, Team Miles is led by the company Fluid and Reason LLC. Team Miles is a group of citizen scientists and engineers that initially came together through Tampa Hackerspace in Florida – all participants in the community, nonprofit workshop. Team Miles is a 6U CubeSat to demonstrate navigation in deep space using innovative plasma thrusters. Use of a software defined S-band radio to communicate with Earth.
NASA has also reserved three slots for payloads from international partners. These are:
• EQUULEUS (EQUilibriUm Lunar-Earth point 6U Spacecraft) of ISSL (Intelligent Space Systems Laboratory) of the University of Tokyo and JAXA. EQUULEUS will help scientists understand the radiation environment in the region of space around Earth by imaging Earth’s plasmasphere and measuring the distribution of plasma that surrounds the planet. This opportunity may provide important insight for protecting both humans and electronics from radiation damage during long space journeys. It will also demonstrate low-energy trajectory control techniques, such as multiple lunar flybys, within the Earth-Moon region.
• OMOTENASHI (Outstanding MOon exploration TEchnologies demonstrated by NAno Semi-Hard Impactor) of JAXA. JAXA will use the OMOTENASHI to demonstrate the technology for low-cost and very small spacecraft to explore the lunar surface. This technology could open up new possibilities for future missions to inexpensively investigate the surface of the moon. The CubeSat will also take measurements of the radiation environment near the moon as well as on the lunar surface.
• ArgoMoon. The Italian company Argotec is building the ArgoMoon CubeSat under the Italian Space Agency (ASI) internal review and approval process. ArgoMoon will demonstrate the ability to perform operations in close proximity of the ICPS ( Interim Cryogenic Propulsion Stage), which will send Orion onto its lunar trajectory. It should also record images of the ICPS for historical documentation and to provide valuable mission data on the deployment of other CubeSats. Additionally, this CubeSat should test optical communication capabilities between the CubeSat and Earth.
All the CubeSats will ride to space inside the OSA (Orion Stage Adapter), which sits between the ICPS (Interim Cryogenic Propulsion Stage) and Orion (Figure 12). The CubeSats will be deployed following Orion separation from the upper stage and once Orion is a safe distance away.
The SPIE ( Spacecraft and Payload Integration and Evolution) office is located at NASA/MSFC (Marshall Space Flight Center) in Huntsville, Alabama, which handles integration of the secondary payloads.
These small satellites are designed to be efficient and versatile—at no heavier than 14 kg, they are each about the size of a boot box, and do not require any extra power from the rocket to function. The science and technology experiments enabled by these small satellites may enhance our understanding of the deep space environment, expand our knowledge of the moon, and demonstrate technology that could open up possibilities for future missions. 20)
A key requirement imposed on the EM-1 secondary payload developers is that the smallsats do not interfere with Orion, SLS or the primary mission objectives. To meet this requirement, payload developers must take part in a series of safety reviews with the SLS Program’s Spacecraft Payload Integration & Evolution (SPIE) organization, which is responsible for the Block 1 upper stage, adapters and payload integration. In addition to working with payload developers to ensure mission safety, the SLS Program also provides a secondary payload deployment system in the OSA (Orion Space Adapter). The deployment window for the CubeSats will be from the time ICPS disposal maneuver is complete (currently estimated to require about four hours post-launch) to up to 10 days after launch. 21)
Deployment windows of secondary payloads: The smallsats manifested on EM-1 will undertake a diverse variety of experiments and technology demonstrations. Seven payloads will be deployed after the ICPS has cleared the first Van Allen Radiation Belt (bus stop 1, Figure 11).
• JAXA, the Japanese Space Agency, will have two smallsats deploy at the first stop: OMOTENASHI will land the smallest lander to date on the lunar surface to demonstrate the feasibility of the hardware for distributed cooperative exploration systems. If this mission is successful, Japan will be the fourth nation to successfully land a mission on the Earth’s moon. The other JAXA payload, EQUULEUS, will fly to a libration orbit around the EML2 (Earth-Moon L2) point and demonstrate trajectory control techniques within the sun-Earth-moon region for the first time by a smallsat.
• Lunar Flashlight is a NASA/JPL ( Jet Propulsion Laboratory) mission that will look for ice deposits and identify locations where resources may be extracted from the lunar surface.
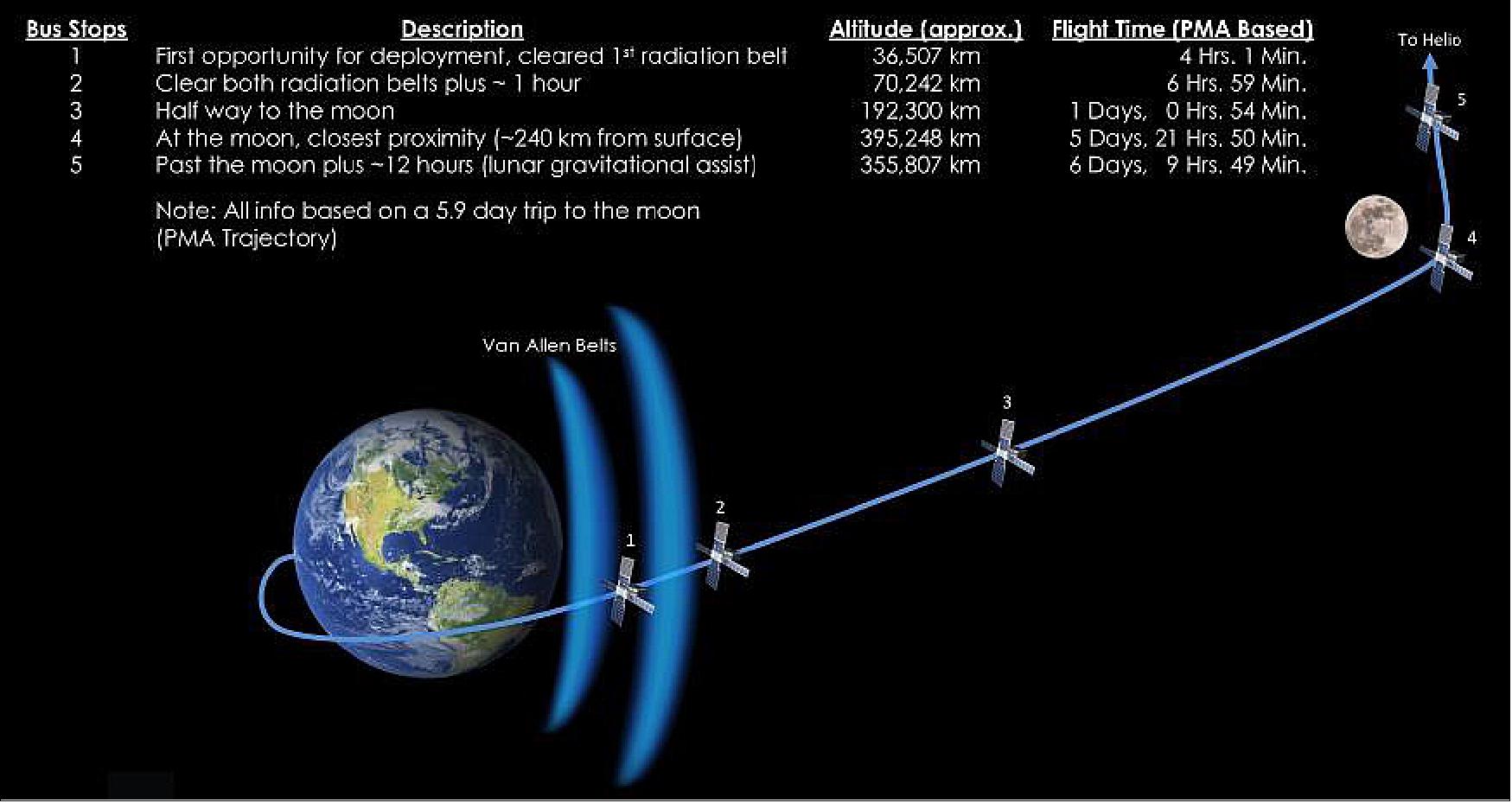
• The NASA/ARC ( Ames Research Center) developed the BioSentinel mission is a yeast radiation biosensor that will measure effects of space radiation on DNA.
• ArgoMoon, sponsored by the Agenzia Spaziale Italiana (ASI), will perform proximity operations with the ICPS post-disposal and record imagery of engineering and historical significance — as well as of the Earth and moon — by testing an advanced software imaging recognition system using high-definition cameras.
• Cislunar Explorers, a team from Cornell University in Ithaca, New York, competing in NASA’s Cube Quest Centennial Challenge competition, has designed a 6U CubeSat that will split into two smaller spacecraft that will orbit the moon using a novel propulsion system of inert water to carry out gravity assists with the moon, and then be captured into lunar orbit.
• Finally, Lunar Icecube, developed by Morehead State University in Kentucky, will search for water in ice, liquid and vapor forms as well as other lunar volatiles from a low-perigee, highly inclined lunar orbit using a compact infrared spectrometer.
• About 90 minutes after the ICPS clears the first Van Allen Belt, the NEA Scout (Near Earth Asteroid) Scout, a NASA/MSFC (Marshall Space Flight Center) mission equipped with a solar sail to rendezvous with an asteroid, will be deployed. NEA Scout will gather detailed imagery and observe the asteroid’s position in space.
• After the ICPS has cleared both radiation belts, the LunaH-Map (Lunar-Polar Hydrogen Mapper) payload from Arizona State University will be released. LunaH-Map will help scientists understand the quantity of hydrogen-bearing materials in cold traps in permanently shaded lunar craters via low-altitude flybys of the moon’s south pole.
• About one hour after clearing the radiation belts (bus stop 2, Figure 11), Lockheed Martin’s LunIR spacecraft, a technology demonstration mission that will perform a lunar flyby, will be deployed. Using a miniature high-temperature MWIR (Mid-Wave Infrared) sensor to collect spectroscopy and thermography data, LunIR will provide data related to surface characterization, remote sensing and site selection for lunar future missions.
About 12 hours after the ICPS passes the moon (bus stop 5, Figure 11) and uses its gravity to enter heliocentric orbit, the final three smallsats will be released.
• The CuSP (CubeSat Mission to Study Solar Particles) mission of SwRI (Southwest Research Institute) in San Antonio, Texas, will study the sources and acceleration mechanisms of solar and interplanetary particles in near-Earth orbit, support space weather research by determining proton radiation levels during ESP (Solar Energetic Particle) events and identifying suprathermal properties that could help predict geomagnetic storms.
• Team Miles, of Miles Space, LLC, of Tampa, Florida, another Cube Quest competitor, has a mission that will fly autonomously using a sophisticated onboard computer system. The spacecraft will be propelled by evolutionary plasma thrusters.
• The final Cube Quest entrant, the University of Colorado CU-E3 (Earth Escape Explorer), is a CubeSat from the University of Colorado in Boulder, Colorado, that will use solar radiation pressure rather than an onboard propulsion system.
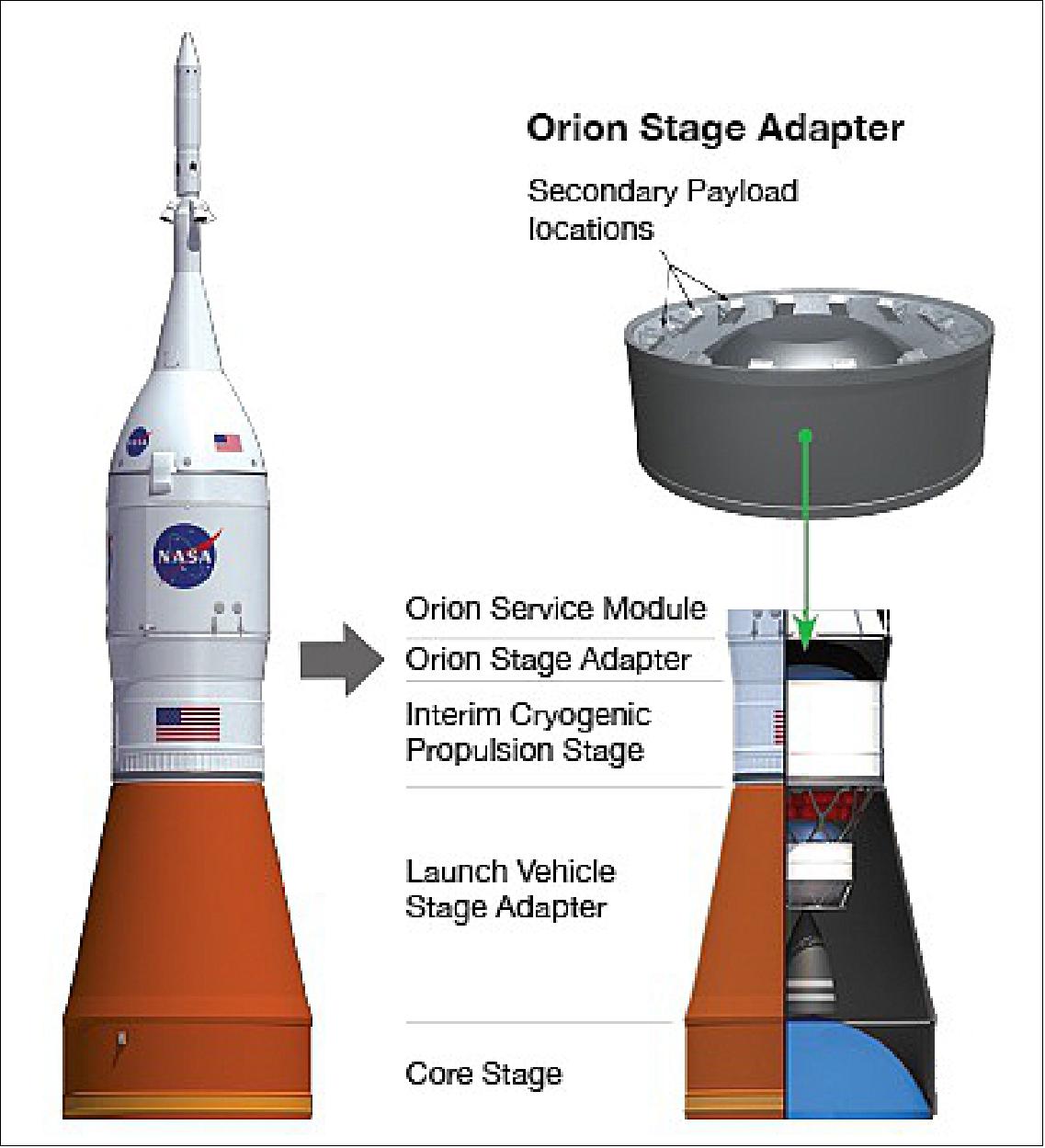
Sensor Complement
BIRCHES (Broadband InfraRed Compact, High-resolution Exploration Spectrometer), a miniaturized version of OVIRS on OSIRIS-REx
BIRCHES is a compact (1.5U, 2.5 kg, 10-15 W including cryocooler) point spectrometer with a compact cryocooled HgCdTe focal plane array for broadband (1 to 4 µm) measurements, achieving sufficient SNR (>400) and spectral resolution (10 nm) through the use of a Linear Variable Filter to characterize and distinguish important volatiles (water, H2S, NH3, CO2, CH4, OH, organics) and mineral bands. The instrument has built-in flexibility, using an adjustable 4-sided iris, to maintain the same spot size regardless of variations in altitude (by up to a factor of 5) or to vary spot size at a given altitude, as the application requires. Compact instrument electronics are also being developed which can be easily reconfigured to support the instrument in ‘imager’ mode, once the communication downlink bandwidth becomes available, and the H1RG family of focal plane arrays (Ref. 4).
Thermal design is critical for the instrument. The compact and efficient Ricor cryocooler is designed to maintain the detector temperature below 120 K. In order to maintain the optical system below 220 K, a special radiator is dedicated to optics alone, in addition to a smaller radiator to maintain a nominal environment for spacecraft electronics.
The BIRCHES instrument has a size of 10 x 10 x 15 mm. The power subsystem requirement of 10-15 W includes the 3 W detector electronics, a 1.5 W AFS controller, and the 5-10 W cryocooler.
BIRCHES observation requirements (Ref. 3):
• A footprint of 10 km from a lunar altitude of 100 km. Footprint of 10 km in along-track direction regardless of altitude, larger in cross-track direction above 250 km
• Nyquist sampling of the lunar surface
• FOV of the instrument is 100 mrad (6º)
• An AFS (Adjustable Field Stop) shall maintain the FOV to 100 km in size
• Based on spacecraft velocity, exposes shall be taken at intervals of 2.7 s (TBC).
Species | Wavelength (µm) | Description |
Water vapor | 2.738 2.663 | OH stretch |
Liquid water | 3.106 | H-OH fundamental |
| 1.4 | OH stretch overtone |
| 2.85 | M3 Feature |
Hydroxyl ion | 2.7-2.8 | OH stretch (mineral) |
| 2.2-2.3 | Cation-OH bend |
Bound H2O | 2.85 | Houck et al. (Mars) |
| 3.14 | Feature w/2.95 |
Adsorbed H2O | 2.9-3.0 | R. Clark |
Ice | 1.5 | Band depth-layer correlated |
| 3.06 | Pieters et al. |
Other volatiles | ||
NH3 | 1.65, 2, 2.2 | N-H stretch |
CO2 | 2, 2.7 | C-O vibration and overtones |
H2S | 3 |
|
CH4/organics | 1.2, 1.7, 2.3, 3.3 | C-H stretch fundamental and overtones |
Mineral Bands | ||
Pyroxene | 0.95-1 | crystal field effects, charge transfer |
Olivine | 1, 2, 2.9 | crystal field effects |
Spinels | 2 | crystal field effects |
Iron oxides | 1 | crystal field effects |
Carbonate | 2.35, 2.5 | overtone bands |
Sulfide | 3 | conduction bands |
Hydrated silicates | 3-3.5 | vibrational processes |
Anticipate wavelength of peak for water absorption band to be structural<bound<adsorbed<ice | ||
Yellow = water-related features in the 3 µm region |
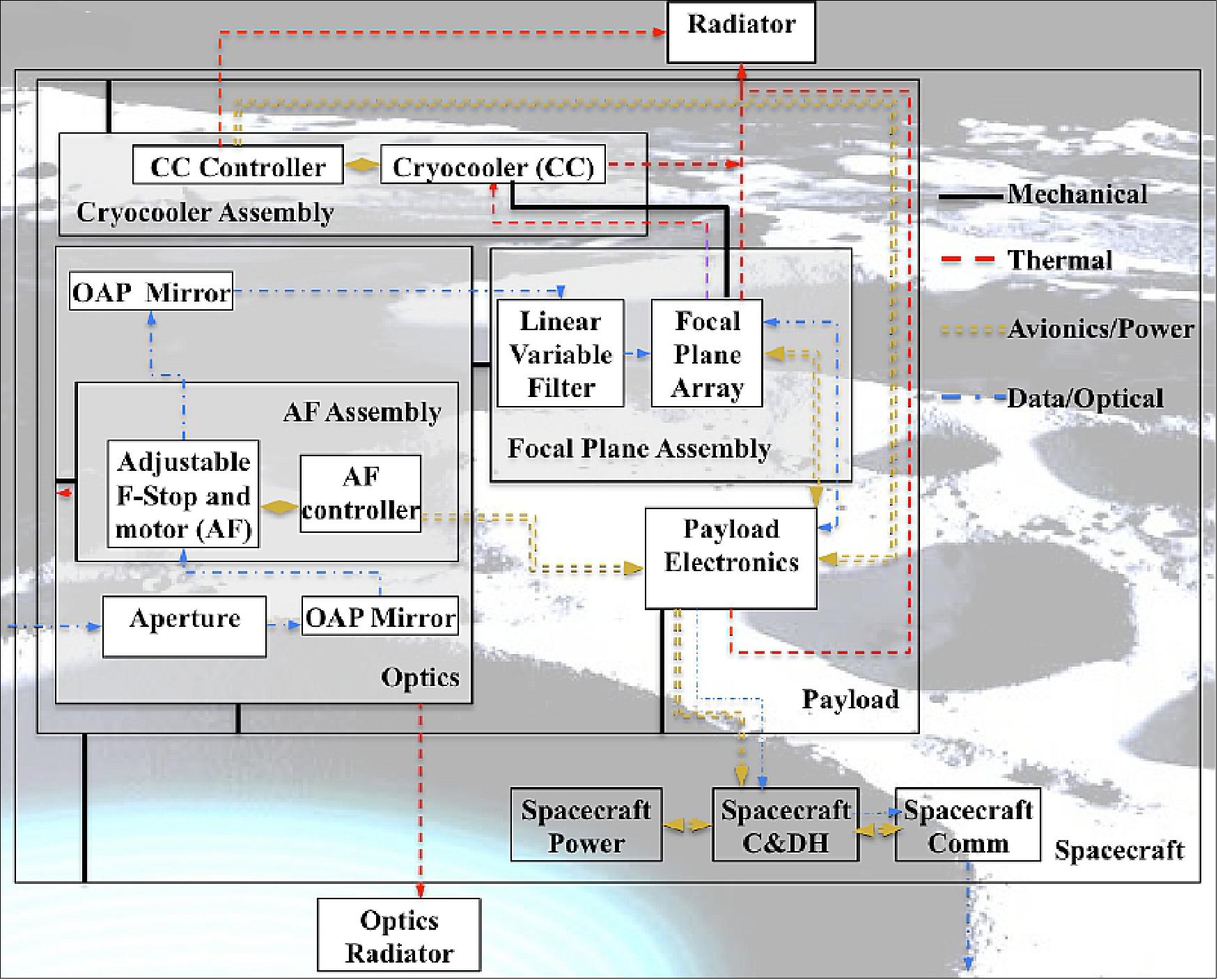
BIRCHES utilizes a compact Teledyne H1RG HgCdTe FPA (Focal Plane Array) and a JDSU (formerly JDS Uniphase Corporation) of Milpitas, CA, LVF (Linear Variable Filter) detector assembly leveraging OSIRIS-REx OVIRS.
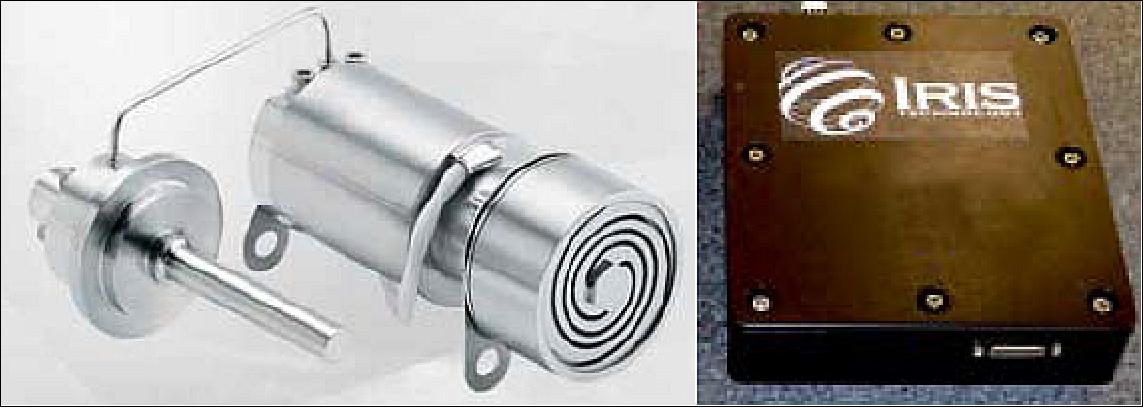
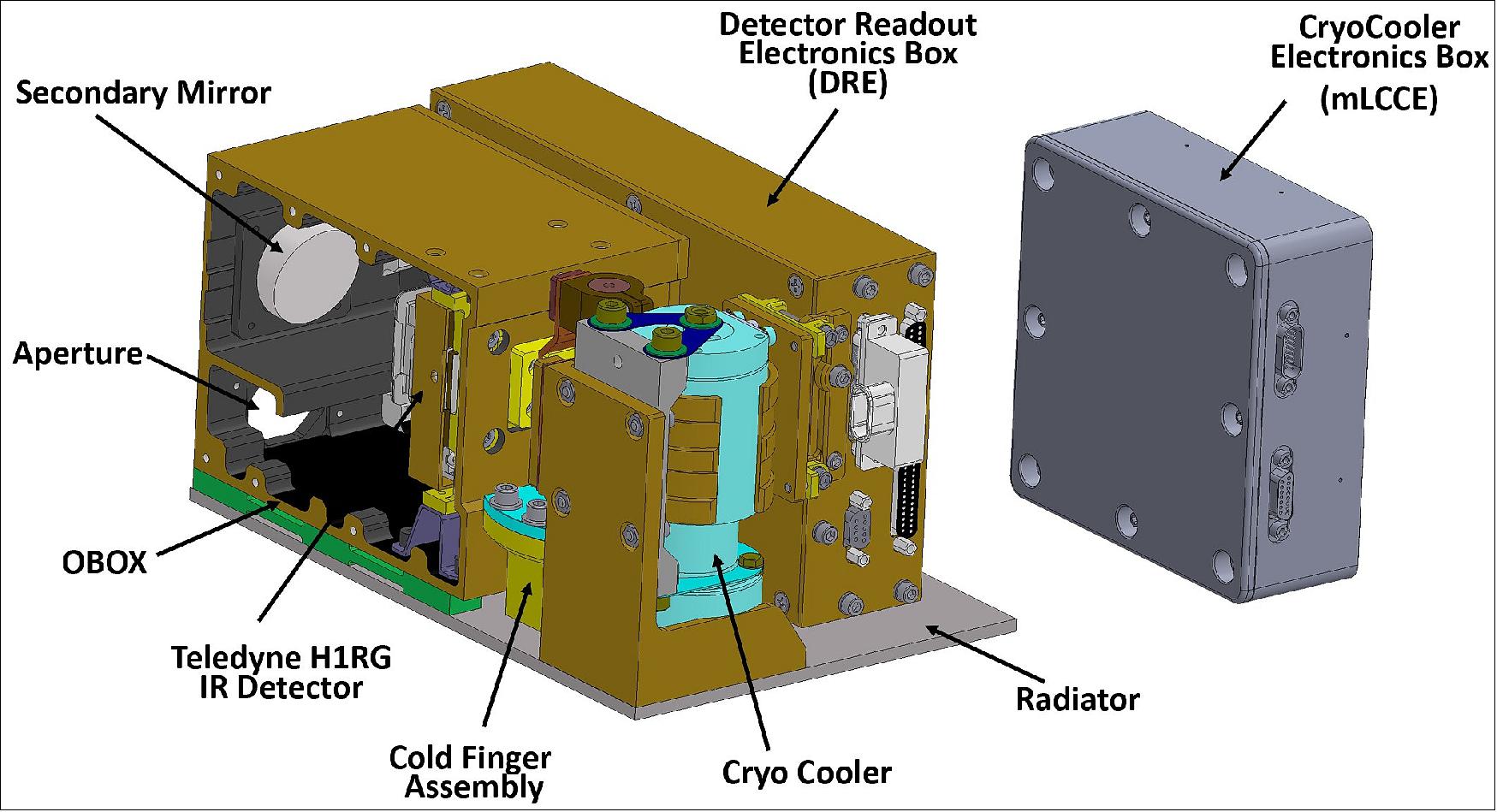
References
1) ”Lunar IceCube to Take on Big Mission From Small Package,” NASA, August 4, 2015 (updated on Jan. 29, 2016), URL: https://www.nasa.gov/feature/
goddard/lunar-icecube-to-take-on-big-mission-from-small-package
2) Michael Tsay, John Frongillo, Kurt Hohman, Benjamin K. Malphrus, ”Linar Cube: A Deep Space 6U CubeSat with Mission Enabling Ion Propulsion Technology,” Proceedings of the 29th Annual AIAA/USU Conference on Small Satellites, Logan, Utah, USA, August 8-13, 2015, paper: SSC15-XI-1, URL: http://digitalcommons.usu.edu/cgi/viewcontent.cgi?article=3235&context=smallsat
3) P. E. Clark, Ben Malphrus, Kevin Brown, Dennis Reuter, Robert MacDowall, David Folta, Avi Mandell, Terry Hurford, Cliff Brambora, Deepak Patel, Stuart Banks, William Farrell, Noah Petro, Michael Tsay, V. Hruby, Carl Brandon, Peter Chapin, ”Lunar Ice Cube Mission: Determining Lunar Water Dynamics with a First Generation Deep Space CubeSat,” 47th Lunar and Planetary Science Conference (2016), The Woodlands, Texas, March 21-25, 2016, paper: 1043.pdf, URL: http://www.hou.usra.edu/meetings/lpsc2016/pdf/1043.pdf
4) Pamela E. Clark, Ben Malphrus, D. Reuter, T. Hurford, R. MacDowall, N. Petro, W. Farrell, C. Brambora, D. Patel, S. Banks, P. Coulter, D. Folta, P. Calhoun, B. Twiggs, Jeff Kruth, Kevin Brown, R. McNeill, M. Tsay, V. Hruby, Carl Brandon, Peter Chapin, ”Broadband InfraRedCompact High-resolution Exploration Spectrometer: Lunar Volatile Dynamics for the Lunar Ice Cube Mission,” Proceedings of the 30th Annual AIAA/USU SmallSat Conference, Logan UT, USA, August 6-11, 2016, URL: http://digitalcommons.usu.edu/cgi/viewcontent.cgi?article=3474&context=smallsat
5) B. Malphrus, K. Z. Brown, P. E. Clark, T. Hurford, D. Folta, C. Brambora, M. Grubb, M. Tsay, “The Lunar IceCube EM-1 Mission: Prospecting for Lunar Water Ice” IEEE Aerospace Electronic Systems, volume 34, number 4, April 2019
6) Benjamin K. Malphrus, Kevin Z. Brown, Jose Garcia, Charles Conner, Jeff Kruth, Michael S. Combs, Nathan Fite, Sean McNeil, Pamela Clark, Krisjani Angkasa, Dave Folta, Cliff Brambora, Paul Mason, Sun Hur-Diaz, Joseph Nakamura, Andres Martinez, Michael Tsay, Matthew Grubb, & Cody Cutright, ”Lunar IceCube: Pioneering Technologies for Interplanetary Small Satellite Exploration,” Proceedings of the 70th IAC (International Astronautical Congress), Washington DC, USA, 21-25 October 2019, paper: IAC-19- 19.B4.8.4, URL: https://iafastro.directory/iac/proceedings/
IAC-19/IAC-19/B4/8/manuscripts/IAC-19,B4,8,4,x51234.pdf
7) Pamela E. Clark, Ben Malphrus, Kevin Brown, Nate Fite, Jacob Schabert, Sean McNeil, Cliff Brambora, Jerrod Young, Deepak Patel, Terry Hurford, David Folta, and Paul Mason "Lunar Ice Cube: ongoing development of first generation deep space CubeSat mission with compact broadband IR spectrometer", Proceedings of SPIE , Vol. 11131, CubeSats and SmallSats for Remote Sensing III, 1113108 (30 August 2019), https://doi.org/10.1117/12.2529323, SPIE Optical Engineering + Applications, 2019, San Diego, California, USA
8) ”Iris V2 CubeSat Deep-Space Transponder (IRIS),” NASA/JPL, URL: https://www.nasa.gov/sites/default/files/atoms/files/brochure_irisv2_201507.pdf
9) Michael Tsay, John Frongillo, Kurt Hohman, ”Iodine-Fueled Mini RF Ion Thruster for CubeSat Applications,” Proceedings of the 34th International Electric Propulsion Conference (IEPC), Hyogo-Kobe, Japan, July 4-10, 2015, paper: IEPC-2015-273, URL: http://erps.spacegrant.org/uploads/images/2015Presentations/IEPC-2015-273_ISTS-2015-b-273.pdf
10) Michael Tsay, John Frongillo, Joshua Model, Jurg Zwahlen,Lenny Paritsky, ”Flight Development of Iodine BIT-3 RF Ion Propulsion System for SLS EM-1 CubeSats,” Proceedings of the 30th Annual AIAA/USU SmallSat Conference, Logan UT, USA, August 6-11, 2016, URL: http://digitalcommons.usu.edu/cgi/viewcontent.cgi?article=3471&context=smallsat
11) James Szabo,Mike Robin, ”Plasma Species Measurements in the Plume of an Iodine Fueled Hall Thruster,” Journal of Propulsion and Power, Vol. 30, No. 5, Sept.2014, pp. 1357-1367, http://dx.doi.org/10.2514/1.B35075
12) Katherine Schauer, ”Lunar IceCube Passes Critical Testing at NASA’s Goddard Space Flight Center,” NASA Feature, 8 June 2021, URL: https://www.nasa.gov/image-feature/goddard/2021/
lunar-icecube-passes-critical-testing-at-nasa-goddard-space-flight-center
13) Philip Sloss, ”EM-1 Update: Making progress, but still behind schedule,” NASA Spaceflight.com, 3 April 2018, URL: https://www.nasaspaceflight.com/2018/04/em-1-update-progress-still-behind-schedule/
14) Pamela Clark, ”CubeSats in Cislunar Space,” Proceedings of the 32nd Annual AIAA/USU Conference on Small Satellites, Logan UT, USA, Aug. 4-9, 2018, paper: SSC18-V-03, URL: https://digitalcommons.usu.edu/cgi/viewcontent.cgi?article=4094&context=smallsat
15) Ben Malphrus, ”A New Era of Planetary Exploration with Small Satellite Platforms,” 4thIAA Conference on University Satellite Missions and CubeSat Workshop, 4-7 December 2017, Rome, Italy, URL: https://www.gaussteam.com/wordpress/wp-content/uploads/
2018/02/IAA-AAS-CU-2017-12-05-BenjaminMalphrus.pdf
16) Kathryn Hambleton, Kim Newton, / Shannon Ridinger, ”NASA Space Launch System’s First Flight to Send Small Sci-Tech Satellites Into Space,” NASA Press Release 16-011, Feb. 2, 2016, URL: http://www.nasa.gov/press-release/
nasa-space-launch-system-s-first-flight-to-send-small-sci-tech-satellites-into-space
17) Christopher Moore, Jitendra Joshi, Nicole Herrmann, ”Deep-Space CubeSats on Exploration Mission-1,” Proceedings of the 68th IAC (International Astronautical Congress), Adelaide, Australia, 25-29 Sept. 2017, paper:IAC-17-B4.8
18) ”Three DIY CubeSats Score Rides on NASA’s First Flight of Orion, Space Launch System,” NASA Release 17-055, 8 June 2017, URL: https://www.nasa.gov/press-release/
three-diy-cubesats-score-rides-on-nasa-s-first-flight-of-orion-space-launch-system
19) Kathryn Hambleton, Kim Henry, Tracy McMahan, ”International Partners Provide Science Satellites for America’s Space Launch System Maiden Flight,” NASA, 26 May 2016 and update of 07 February 2018, URL: https://www.nasa.gov/exploration/systems/sls/
international-partners-provide-cubesats-for-sls-maiden-flight
20) ”Smallsats Of Scientific Persuasions To Be Supplied By International Partners To NASA For The Maiden Flight Of SLS,” Satnews Daily, May 31, 2016, URL: http://www.satnews.com
/story.php?number=1735749470
21) Kimberly F. Robinson, Scott F. Spearing, David Hitt, ”NASA’s Space Launch System: Opportunities for Small Satellites to Deep Space Destinations,” Proceedings of the 32nd Annual AIAA/USU Conference on Small Satellites, Logan UT, USA, Aug. 4-9, 2018, paper: SSC18-IX-02, URL: https://digitalcommons.usu.edu/cgi/viewcontent.cgi?article=4119&context=smallsat
The information compiled and edited in this article was provided by Herbert J. Kramer from his documentation of: ”Observation of the Earth and Its Environment: Survey of Missions and Sensors” (Springer Verlag) as well as many other sources after the publication of the 4th edition in 2002. - Comments and corrections to this article are always welcome for further updates (eoportal@symbios.space).
Spacecraft Launch Secondary Payloads Sensor Complement References Back to top