GAIA Astrometry Mission
Non-EO
ESA
Operational (extended)
ASTRIUM
Quick facts
Overview
Mission type | Non-EO |
Agency | ESA, ASTRIUM |
Mission status | Operational (extended) |
Launch date | 19 Dec 2013 |
Gaia Astrometry MissionSpacecraft Launch Mission Status Payload Module Ground Segment Big Data Archive References
Gaia (mother Earth in Greek mythology) is an ESA cornerstone space astrometric mission, part of the Horizon 2000 Plus long-term scientific program, with the goal to compile a 3D space catalog of > 1000 million stars, or roughly 1% of the stars in our home galaxy, the Milky Way. Gaia will monitor each of its target stars about 70 times to a magnitude of G=20 over a period of 5 years. It will precisely chart their positions, distances, movements, and changes in brightness. It is expected to discover hundreds of thousands of new celestial objects, such as extra-solar planets and brown dwarfs, and observe hundreds of thousands of asteroids within our own Solar System. The mission will also study about 500,000 distant quasars and will provide stringent new tests of Albert Einstein’s General Theory of Relativity. 1) 2) 3) 4) 5)
Cataloguing the night sky is an essential part of astronomy. Before astronomers can investigate a celestial object, they must know where to find it. Without this knowledge, astronomers would wander helplessly in what Galileo once termed a ‘dark labyrinth’.
During the satellite’s expected lifetime of five years, Gaia will observe each star about 70 times, each time recording its brightness, color and, most importantly, its position. The precise measurement of a celestial object’s position is known as astrometry, and since humans first started studying the sky, astronomers have devoted much of their time to this art. However, Gaia will do so with extraordinary precision, far beyond the dreams of those ancient astronomers.
By comparing Gaia’s series of precise observations, today’s astronomers will soon be able to make precise measurements of the apparent movement of a star across the heavens, enabling them to determine its distance and motion through space. The resulting database will allow astronomers to trace the history of the Milky Way.
In the course of charting the sky, Gaia’s highly superior instruments are expected to uncover vast numbers of previously unknown celestial objects, as well as studying normal stars. Its expected haul includes asteroids in our Solar System, icy bodies in the outer Solar System, failed stars, infant stars, planets around other stars, far-distant stellar explosions, black holes in the process of feeding and giant black holes at the centers of other galaxies.
The primary mission objectives are:
• Measure the positions and velocity of approximately one billion stars in our Galaxy
• Determine their brightness, temperature, composition and motion through space
• Create a three-dimensional map of the Galaxy.
Additional discoveries expected:
- hundreds of thousands of asteroids and comets within our Solar System
- seven thousand planets beyond our Solar System
- tens of thousands of ‘failed’ stars, called brown dwarfs
- twenty thousand exploding stars, called supernovae
- hundreds of thousands of distant active galaxies, called quasars.
The Gaia objective is to provide a very accurate dynamical 3D map of our Galaxy by using global astrometry from space, complemented with multi-color multi-epoch photometric measurements. The aim is to produce a catalog complete for star magnitudes up to 20, which corresponds to more than one billion stars or about 1% of the stars of our Galaxy. The instrument sensitivity is such that distances beyond 20-100 kiloparsec (kpc) will be covered, therefore including the Galaxy bulge (8.5 kpc) and spiral arms. The measurements will not be limited to the Milky Way stars. These include the structure, dynamics and stellar population of the Magellanic Clouds, the space motions of Local Group Galaxies and studies of supernovae, galactic nuclei and quasars, the latter being used for materializing the inertial frame for Gaia measurements.

Background
Gaia is ESA's second space mission dedicated to astrometry. It builds on the legacy of the successful Hipparcos mission (1989-1993). 7) Like Hipparcos, Gaia's observation strategy is based on detecting stellar positions in two fields of view separated by a 'basic angle', which for Gaia is 106.5º. This strategy allows astronomers to establish a coherent reference frame over the entire sky, yielding highly accurate measurements of stellar positions.
After a detailed concept and technology study during 1998–2000, Gaia was selected as a confirmed mission within ESA’s scientific program in October 2000. It was confirmed by ESA’s Science Program Committee following a re-evaluation of the science program in June 2002, and reconfirmed following another re-evaluation of the program in November 2003. The project entered Phase-B2/C/D in February 2006. As of the summer 2012, Gaia is in Phase-D (Qualification and Production) and will be launched in the second half of 2013. 8) 9) 10)
• In June 2013, ESA's billion-star surveyor, Gaia, has completed final preparations in Europe and is ready to depart for its launch site in French Guiana. The Gaia spacecraft arrived in Cayenne, French Guiana, on August 23, 2013 on board the Antonov 124 aircraft.
• On Oct. 23, 2013, ESA postponed the launch of the Gaia mission. The decision was taken due to a technical issue that was identified in another satellite already in orbit. The issue concerns components used in two transponders on Gaia that generate ‘timing signals’ for downlinking the science telemetry. To avoid potential problems, they will be replaced.
The transponders were removed from Gaia at Kourou and returned to Europe, where the potentially faulty components were replaced and verified. After the replacements have been made, the transponders will be refitted to Gaia and a final verification test made. As a consequence of these precautionary measures, it will not be possible to launch Gaia within the window that includes the previously targeted launch date of 20 November. The next available launch window is 17 December to 5 January 2014. 11)
• Update Oct. 20, 2013: The upcoming launch manifest of Arianespace has now been established. Gaia is scheduled for launch on 20 December.
• Update Nov. 22, 2013: The checks on the Gaia satellite are proceeding well, enabling the launch to take place on December 19, 2013 (Ref. 11).
Some Astrometry Basics
The precise measurement of a celestial object’s position is known as astrometry, and since humans first started studying the sky, astronomers have devoted much of their time to this art. However, Gaia will do so with extraordinary precision, far beyond the dreams of those ancient astronomers (Ref. 21). 12)
By comparing Gaia’s series of precise observations, today’s astronomers will soon be able to make precise measurements of the apparent movement of a star across the heavens, enabling them to determine its distance and motion through space. The resulting database will allow astronomers to trace the history of the Milky Way.
In the course of charting the sky, Gaia’s highly superior instruments are expected to uncover vast numbers of previously unknown celestial objects, as well as studying normal stars. Its expected haul includes asteroids in our Solar System, icy bodies in the outer Solar System, failed stars, infant stars, planets around other stars, far-distant stellar explosions, black holes in the process of feeding and giant black holes at the centers of other galaxies. Gaia will be a discovery machine.
Stars as Individuals and Collectives
To understand fully the physics of a star, its distance from Earth must be known. This is more difficult than it sounds because stars are so remote. Even the closest one is 40 trillion km away, and we cannot send spacecraft out to them to measure as they go. Nor can we bounce radar signals off them, which is the method used to measure distances within the Solar System. Instead, astronomers have developed other techniques for measuring and estimating distances.
The most reliable and only direct way to measure the distance of a star is by determining its 'parallax'. By obtaining extremely precise measurements of the positions of stars, Gaia will yield the parallax for one billion stars; more than 99% of these have never had their distances measured accurately. Gaia will also deliver accurate measurements of other important stellar parameters, including the brightness, temperature, composition and mass. The observations will cover many different types of stars and many different stages of stellar evolution.

The Principles of Gaia
At its heart, Gaia is a space telescope – or rather, two space telescopes that work as one. These two telescopes use ten mirrors of various sizes and surface shapes to collect, focus and direct light to Gaia’s instruments for detection. The main instrument, an astrometer, precisely determines the positions of stars in the sky, while the photometer and spectrometer spread their light out into spectra for analysis.
Gaia’s telescopes point at two different portions of the sky, separated by a constant 106.5º. Each has a large primary mirror with a collecting area of about 0.7 m2. On Earth we are used to round telescope mirrors, but Gaia’s will be rectangular to make the most efficient use of the limited space within the spacecraft. These are not large mirrors by modern astronomical standards, but Gaia’s great advantage is that it will be observing from space, where there is no atmospheric disturbance to blur the images. A smaller telescope in space can yield more accurate results than a large telescope on Earth.
Gaia is just 3.5 m across, so three curved mirrors and three flat ones are used to focus and repeatedly fold the light beam over a total path of 35 m before the light hits the sensitive, custom-made detectors. Together, Gaia’s telescopes and detectors will be powerful enough to detect stars up to 400,000 times fainter than those visible to the naked eye.
Gaia uses the global astrometry concept demonstrated by Hipparcos. The principle is to link stars with large angular distances in a network where each star is connected to a large number of other stars in every direction. The condition of closure of the network ensures the reduction of the position errors of all stars. This is achieved by the simultaneous observation of two fields of views separated by a very stable basic angle. The spacecraft is slowly rotating at a constant angular rate of 1º/min around a spin axis perpendicular to both fields of view, which describe a great circle on the sky in 6 hours. The spacecraft rotation axis makes an angle of 45º with the Sun direction (Figure 3). A slow precession around the Sun-to-Earth direction, with a 63.12 days period, enables to repeat the observation of sky objects with 86 transits on average over the 5 years of mission.

The resulting performance will enable a breakthrough in the astrometry field, as well regarding star position and velocity performance as for the number of objects observed.

Spacecraft
Gaia is an exceptionally complex space observatory. ESA awarded Airbus Defence and Space (former Astrium SAS,Toulouse, France) the prime contract in May 2006 to develop and build the spacecraft. Together with the German and British branches of Astrium, more than 50 industrial subcontractor companies from across Europe are involved in building this discovery machine. The Gaia DPAC (Data Processing and Analysis Consortium) will process the raw data to be published in the largest stellar catalog ever made. 13) 14) 15) 16) 17) 18) 19) 20)
The Gaia spacecraft is composed of two sections: the Payload Module and the Service Module. The Payload Module is housed inside a protective dome and contains the two telescopes and the three science instruments. They are all mounted on a torus made of a ceramic material (silicon carbide). The extraordinary measurement accuracy required from Gaia calls for an extremely stable Payload Module that will barely move or deform once in space; this is achieved thanks to the extensive use of this material. 21)
Underneath the Payload Module, the Service Module contains electronic units to run the instruments, as well as the propulsion system, communications units and other essential components. These components are mounted on CFRP (CarbonFiber Reinforced Plastic) panels in a conical framework.
Finally, beneath the Service Module, a large sunshield keeps the spacecraft in shadow, maintaining the Payload Module at an almost constant temperature of around -110ºC, to allow the instruments to take their precise and sensitive readings. The sunshield measures about 10 m across, too large for the launch vehicle fairing, so it comprises a dozen folding panels that will be deployed after launch. Some of the solar array panels that are needed to generate power are fixed on the sunshield, with the rest on the bottom of the spacecraft.
The Gaia spacecraft configuration is driven by the required very high thermo-mechanical stability of the entire spacecraft. A low disturbance cold gas micro-propulsion is used for fine attitude control. The astrometric instrument is used for precise rate sensing in fine pointing operating mode.
Spacecraft launch mass | ~2034 kg (dry mass = 1392 kg) |
Spacecraft power | 1.91 kW (EOL) |
Spacecraft dimensions | Height = 3.5 m, deployed diameter = 10 m |
Mission duration | 5 years |

SVM (Service Module)
The SVM is generally referred to as 'the platform'. The SVM in turn is comprised of MSM (Mechanical Service Module) and an ESM (Electrical Service Module). 22) 23) 24)
MSM (Mechanical Service Module): The spacecraft main structure is of hexagonal conical shape. It is a sandwich panel structure with CFRP (Carbon Fiber Reinforced Plastic) face sheets, and a central cone supporting the propellant tanks. The MSM houses instruments needed for the basic control and operation of the satellite; this includes all mechanical, structural and thermal elements that support the instrument payload and spacecraft electronics. It also includes the chemical & micro propulsion systems, the deployable sunshield with solar arrays, the payload thermal tent and harness. The module consists of a central tube that is about 1.17 m long and hosts six radial panels to create a hexagonal spacecraft shape.
The service module also houses the communication subsystem, central computer and data handling subsystem, the high rate data telemetry, attitude control and star trackers. For telemetry and telecommand, low gain antenna uplink and downlink with a few kbit/s capacity are employed. The high gain antenna used for the science telemetry downlink will be used during each ground station visibility period of an average of about 8 hours per day.

ESM (Electrical Service Module): The ESM design is driven by the science performance (attitude control laws with the hybridization of star tracker and payload measurements, high rate data telemetry, and regulated power bus for thermal stability). It houses the AOCS units, the communication subsystem, central computer and data handling subsystem, and the power subsystem.

AOCS (Attitude and Orbit Control Subsystem). The AOCS subsystem is characterized by:
- High precision 3-axis control
- The ASTRO (Astrometric) instrument is used for precise rate sensing during the fine pointing operational mode
- A high precision gyroscope is used for quick and efficient transitions during the fine pointing operational mode. Three FOGs (Fiber Optics Gyroscopes) use the interference of light to detect mechanical rotation. Each unit contains four closed-loop gyroscope channels to provide built-in redundancy.
- Rugged flight-proven initial acquisition and safe modes
- Three sun acquisition sensors plus one gyroscope provide spin-axis stabilization during the L2 transfer phase of the mission
- One large field of view star sensor plus use of the main instrument SM (Sky Mapper) for the 3-axis controlled operational phase.
Gaia AOCS architecture is based on a fully redundant set of equipment. Moving parts on board are strictly minimized (e.g. no reaction wheels, no mechanically steerable antenna). The data is downlinked through a novel electromagnetically steerable phased array antenna and attitude control is provided by a micro propulsion system that has its first flight use with Gaia. An atomic clock is used for precise time-stamping.
Two Autonomous Star Trackers are used in cold redundancy three FSS (Fine Sun Sensors) are used in hot redundancy through triple majority voting. Three Gyro packages provide coarse rate measurements where each gyro package comprises two fully independent co-aligned channels (i.e. a fiber optic gyroscope sensor plus associated electronics per channel), with the channels being used in cold redundancy. As mentioned above, the payload module provides very precise rate measurements when the spacecraft is operated in fine pointing science modes. 25)
For actuators, a bi-propellant Chemical Propulsion System (2 x 8 10 N thrusters used in cold redundancy) is used for orbit maintenance and attitude control in coarse AOCS modes (circa 350 kg MON + MMH).
The Micro-propulsion System (2 x 6 proportional micro-thrusters) can provide a range of 0 - 1000 µN at a resolution of 0.1 µN. The individual thrusters are driven by the micro-propulsion electronic, which is internally redundant and used in cold redundancy (circa 57 kg of GN2). The nominal science AOCS mode uses the cold gas micro-propulsion system.
C&DMS (Command & Data Management Subsystem). The C&DMS is characterized by:
- An ERC-32 based central computer and distinct input/output units for efficient software development
- Two segregated MIL-STD-1553 B data buses: one for the payload module and one for the service module
- SpaceWire data links for high-speed payload data
- FDIR architecture aiming at preserving payload integrity, with built-in autonomy for increased availability.
The PDHU (Payload Data Handling Unit) is, among other things, the 'hard-disk' of Gaia, responsible for temporary storage of science data received from the telescope before transmission back to Earth. It will receive thousands of compressed images per second from the observing system; this data will be sorted and stored. The individual star data objects will be prioritized based on the magnitude of the star. A complex file management system allows deletion of low-priority data in the event of data rates or volumes that exceed the capacity of the storage or transmission systems.
The solid-state storage subsystem of the PDHU has a capacity of 960 GB which, while not impressive by terrestrial standards, is extremely large for a space system. It uses a total of 240 SDRAM modules, each with a capacity of 4 GB, which populate six memory boards. The PDHU controller board is responsible for communication with the other spacecraft subsystems, file system management and the management of telemetry and telecommands. 26) 27)

The PDHU communicates with the gigapixel focal plane over seven redundant 40 Mbit/s SpaceWire channels to acquire the scientific data coming from the seven VPUs (Video Processing Units) of the camera. The unit's controller sorts the incoming data according to star magnitude and manages deletion of low priority data should this become necessary. It sends data for transmission to Earth under the control of the CDMU (Command and Data Management Unit). The PDHU communicates with the CDMU via a MIL-STD-1553 data bus and delivers the science data over two 10 Mbit/s PacketWire channels.- The PDHU consumes only 26 W, has a mass of 14 kg, and occupies a volume of 2.3 liter.
EPS (Electrical Power Subsystem): The spacecraft is equipped with a 12.8 m2 high-efficiency triple-junction GaAs (Gallium-Arsenide) cell solar array, of which 7.3 m2 is in the form of a fixed solar array and 5.5 m2 is covered by 6 panels mechanically linked to deployable sunshield assembly.
For the launch, the deployable sunshield is folded against the payload module. After separation from the launch vehicle, it is deployed around the fixed solar array, in the same plane. During LEOP (Launch and Early Operations Phase), power is supplied by a 60 Ah mass-efficient Lithium-ion battery.
Optimum power supply during all phases of the mission is ensured by a PCDU (Power Control and Distribution Unit) with maximum power point tracking. The PCDU performs power management by generating a 28 V primary power bus that supplies power to all spacecraft subsystems. It also controls the battery state of charge and generates pyrotechnic commands as well as heater actuation as commanded by the C&DMS (Command & Data Management Subsystem).

Propulsion: After injection into the L2 transfer orbit by the Soyuz-Fregat launcher, a chemical bi-propellant propulsion system (8 x 10 N) is used for the transfer phase. It will cover attitude acquisition, spin control, mid-course corrections, L2 orbit injection, and safe mode.
After arriving at L2, one redundant set of micro-propulsion thrusters will control the spin and precession motion of the spacecraft. Regular orbit maintenance will be performed by using the chemical propulsion thrusters. - The spacecraft uses a cold gas micropropulsion system for fine attitude control.
CPS (Chemical Propulsion Subsystem): CPS is a bi-propellant system using two tanks of Herschel/Planck heritage filled with with a total of ~400 kg of propellant featuring a blowdown ratio of 4:3. Use of monomethylhydrazine as fuel and nitrogen tetroxide as oxidizer. The 10 N thrusters are manufactured by Astrium consisting of a platinum alloy combustion chamber and nozzle that tolerates the operational temperature of 1,500°C. The thruster can be operated in a thrust range of 6 to 12.5 N with a nominal thrust of 10 N which generates a specific impulse of 291 seconds.
MPS (Micro Propulsion Subsystem): The MPS is being used for fine attitude pointing and spin rate management. A total of 12 cold gas thrusters are installed on the spacecraft being grouped in three clusters each featuring four cold gas thrusters. The thruster system uses high-pressure nitrogen propellant to provide very small impulses with a thrust range of 1 - 500 µN. The system uses two nitrogen tanks, each containing 28.5 kg of N2, stored at a pressure of 310 bar (Ref. 19). The CG-MPS (Cold Gas-Micro Propulsion Subsystem) was developed by TAS-I and Selex ES S.p.A., Italy. 28)
RF communications: All communication with the Gaia spacecraft is done using the X-band. For TT&C (Tracking Telemetry and Command), a low gain antenna uplink and downlink with a few kbit/s capacity and an omnidirectional coverage are employed. The science telemetry X-band downlink is based on a set of electronically-scanned phased array antennae accommodated on the service module bottom panel. This high gain antenna is used during each ground station visibility period of about 8 hours per day.
The X-band payload downlink rate is 10 Mbit/s from L2. To achieve this, Gaia uses a specially designed on-board phased array antenna to beam the payload data to Earth (a conventional steerable antenna would disturbed the very precise measurements).
Gaia is equipped with a total of three communication antennas – two LGAs (Low Gain Antennas) and a single X-band Medium Gain Phased Array Antenna. One LGA is located pointing in the +X direction while the other points to –X being located on the Thermal Tent and the base of the spacecraft, respectively. The two LGAs build an omni-direction communications system for housekeeping telemetry downlink and command uplink with data rates of a few kbit/s.
The MGA (Medium Gain Antenna) is located on the base of the Payload Module, protruding the DSA(Deployable Sunshield Assembly) . This directional antenna can achieve data rates of up to 10 Mbit/s for science data and telemetry downlink and telecommand reception.
Demodulation of the uplink signal is completed by the transponder units before the data flow is passed on to the CDMU (Command & Data Management Unit). The downlink data is encoded by the CDMU and modulated in X-band within the transponders before being amplified by the SSPA (Solid State Power Amplifier). The signal is combined in the phased array of the active antenna in order to orient the beam towards the Earth.

CCSDS Image Data Compression ASIC: In order to transmit all the data generated on board, a particularly challenging compression factor averaging 2.8 was necessary. Unfortunately the standard suite of algorithms was not able to reach this target, because of the peculiarities of Gaia imagery, which include ‘outliers’, such as bright stars and planets, and which are marred by the momentary ‘hot pixels’ due to cosmic rays in deep space. Instead, with the support of ESA compression experts, industry developed an ad hoc solution, enabling all Gaia mission data to reach their home planet. 29)
CWICOM (CCSDS Wavelet Image COMpression ASIC) is a very high-performance image compression ASIC that implements the CCSDS 122.0 wavelet-based image compression standard, to output compressed data according to the CCSDS output source packet protocol standard. This integrated circuit was developed by Airbus DS through an ESA contract.
CWICOM offers dynamic, large compressed-rate range and high-speed image compression potentially relevant for compression of any 2D image with bi-dimensional data correlation (such as a hyperspectral data cube). Its highly optimized internal architecture allows lossless and lossy image compression at very high data rates (up to 60 Mpixels/s) without any external memory by taking advantage of its on-chip memory – almost 5 Mbit of embedded internal memory).

CWICOM is implemented using the largest matrix of the Atmel ATC18RHA ASIC family, and is provided within a standard surface mount package (CQFP 256). CWICOM offers a low-power, cost-effective and highly integrated solution for any image compression application, performing CCSDS image compression treatments without requiring any external memory. The simplicity of such a standalone implementation is achieved thanks to a very efficient internal embedded memory organization that removes any need for extra memory chip procurement and the potential obsolescence threatened by being bound to a specific external memory interface.
Change in Data Compression Implementation
In order to transmit all the data generated on board, a particularly challenging compression factor averaging 2.8 was necessary. Unfortunately the standard suite of algorithms [which includes CCSDS standards, including the 122.0 implemented by CWICOM] was not able to reach this target, because of the peculiarities of Gaia imagery, which include ‘outliers’, such as bright stars and planets, and which are marred by the momentary ‘hot pixels’ due to cosmic rays in deep space. Instead, with the support of ESA compression experts, industry developed an ad hoc solution,enabling all Gaia mission data to reach their home planet.
Instead of CWICOM, Gaia applies a tailored data compression algorithm (using a heavily tailored pre-processing stage followed by a variant of the Rice coder), using a software implementation running on VPUs (Video Processing Units). The GOCA (Gaia Optimum Compression Algorithm) project was entrusted by ESA to GTD System & Software Engineering (project prime) and IEEC (Institut d'Estudis Espacials de Catalunya), scientific partner), aimed in providing a deep understanding of the Gaia compression problem and offering a complete data compression system, both at an algorithm and implementation level. The main objectives of GOCA not only encompassed the review and evaluation of the already proposed compression scheme but the design of new algorithms for the mission. 30) 31) 32)
The CCDs in the focal plane are commanded by video-processing units (VPUs). Gaia has seven identical VPUs, each one dealing with a dedicated row of CCDs. Each CCD row, contains in order, two SM CCDs (one for each telescope), 9 AF CCDs, 1 BP CCD, 1 RP CCD, and 3 RVS CCDs (the latter only for four of the seven CCD rows). The VPUs run seven identical instances of the video-processing algorithms (VPAs), not necessarily with exactly the same parameter settings though. This (mix of some hardware and mostly) software is responsible for object detection (after local background subtraction), object windowing (see below), window conflict resolution, data binning, data prioritization, science-packet generation, data compression, etc.) 33)
TCS (Thermal Control Subsystem): A deployable sunshield with optimal thermoelastic behavior, made of multi-layer insulation sheets, is attached to the service module and folded against the payload module for the launch. After separation of the Gaia spacecraft from the launch vehicle, the Sun shield is deployed around the fixed solar array, in the same plane. - A thermal tent covers the payload, offering extra protection against micrometeoroids and radiation.
The very high stability thermal control is mostly passive and is achieved through optical surface reflector material, multilayer insulation sheets on the outer faces of the service module, and a black painted cavity, supplemented by heaters where required. Thermal stability is guaranteed by a constant solar aspect angle and the avoidance (as far as possible) of any equipment switch-ON/OFF cycles during nominal operation.
DSA (Deployable Sunshield Assembly): The bottom floor of the SVM is a dodecagonal-shaped panel to comply with the 12 frames of the DSA The main structure consists of carbon-fiber reinforced plastic face sheets.
DSA is folded up during launch and is deployed early in the flight. It is required to shade the payload unit and protect it from direct sunlight that could compromise instrument accuracy. Keeping the instrument at a constant temperature prevents expansion and contraction during temperature variations which would alter the instrument geometry ever so slightly with a large effect on data quality. The DSA is 10 m in diameter.
The DSA is an umbrella-type structure that consists of MLI (Multilayer Insulation) as the primary shield material and six rigid deployment booms as well as six secondary stiffeners. These booms have a single articulation on the base of the Service Module for easy deployment in the radial direction by a spring system. Spacing cables link the booms to the others to ensure a synchronized deployment sequence. The booms and strings are located on the cold side of the cover to limit thermoelastic flexing.
Attached to the DSA are six rectangular solar panels (with triple-junction solar GaAs cells) that are constantly facing the sun once the shield is deployed. They provide 1910 W of EOL (End of Life) power.

Legend to Figure 12: The DSA during deployment testing at Astrium Toulouse. Since the DSA will operate in microgravity, it is not designed to support its own weight in the one-g environment at Earth's surface. During deployment testing, the DSA panels are attached to a system of support cables and counterweights that bears their weight, preventing damage and providing a realistic test environment. The flight model thermal tent is visible inside the deploying sunshield and the mechanically representative dummy payload can be seen through the aperture in the tent.


Propulsion systems | - 8 x 10 N bipropellant thrusters for coarse attitude control, orbit injection and trajectory corrections |
Command and data management system | - ERC 32 based central computer with embedded solid state mass memory, complemented by a distinct input / output management unit |
3-axes AOCS (Attitude and Orbit Control Subsystem) | - Payload instrument used for precise rate sensing in science mode |
TT&C (Telemetry, Tracking and Command Subsystem) | - All X-band system |
EPS (Electrical Power Subsystem) | - 60 Ah Li-ion battery |
Payload data handling | - 7 Video Processing Units in parallel, each provided with 1 GIPS (Giga Instruction/s) processing capability |
TCS (Thermal Control Subsystem) | - Fully passive thermal control |
Structure | - All Silicium Carbide instrument: structure & mirrors |




Launch
The Gaia spacecraft was launched on December 19, 2013 (09:12:19 UTC) from Kourou by Arianespace, Europe’s Spaceport in French Guiana. The launch vehicle was a Soyuz-STB with a Fregat-MT upper stage The launch is designated as Soyuz flight VS06. 35) 36) 37)
- About ten minutes later, after separation of the first three stages, the Fregat upper stage ignited, delivering Gaia into a temporary parking orbit at an altitude of 175 km.
- A second firing of the Fregat 11 minutes later took Gaia into its transfer orbit, followed by separation from the upper stage 42 minutes after liftoff. Ground telemetry and attitude control were established by controllers at ESOC (European Space Operation Centre) in Darmstadt, Germany, and the spacecraft began activating its systems.
- The sunshield, which keeps Gaia at its working temperature and carries solar cells to power the satellite, was deployed in a 10 minute automatic sequence, completed around 88 minutes after launch. Gaia is now en route towards L2 (Ref. 35).
Orbit: Large Lissajous orbits around L2 (Lagrangian Point 2), about 1.5 million km from Earth. L2 offers a stable thermal environment because the sunshield will protect Gaia from the Sun, Earth and Moon simultaneously, allowing the satellite to keep cool and enjoy a clear view of the Universe from the other side. In addition, L2 provides a moderate radiation environment, which benefits the longevity of the instrument detectors.
• The critical LEOP (Launch and Early Orbit Phase) will last approximately four days. In this phase, Gaia will perform the first activations – transmitter switch ON, priming of the chemical thrusters, first attitude control and finding of the sun position – followed by the sun shield deployment. Engineers on ground will perform orbit determination, then prepare and execute the critical 'Day 2' maneuver to inject Gaia into its final transfer trajectory toward the L2 Lagrange point (Ref. 94).
• LEOP will be followed by the transfer cruise phase, lasting up to 30 days, an L2 orbit injection maneuver, then the in-orbit commissioning phase, during which all operations to prepare for the routine operational phase are performed. In particular, the scientific FPA (Focal Plane Assembly) and related avionics will be thoroughly tested and calibrated. The commissioning phase is expected to last four months.
• The insertion into the final 300,000 x 200,000 km Lissajous orbit around L2 was performed one month after launch.

Note: As of 3 March 2020, the previously single large Gaia file has been split into two files, to make the file handling manageable for all parties concerned, in particular for the user community.
• This article covers the Gaia mission and its imagery in the period 2020, in addition to some of the mission milestones.
• Gaia status and imagery in the period 2019-2013
Mission Status for the Period 2022 - 2020
• June 13, 2022: Since its launch in 2013 ESA’s Gaia observatory has been mapping our galaxy from Lagrange point 2, creating the most accurate and complete multi-dimensional map of the Milky Way. By now two full sets of data have been released, the first set in 2016 and a second one in 2018. These data releases contained stellar positions, distances, motions across the sky, and colour information, among others. Now on 13 June 2022 a third and new full data set will be released. 38)
- This video includes interviews with:
a) Frederic Arenou, CNRS Research Engineer & Gaia Collaboration Scientist, Observatoire de Paris – PSL (in English & French)
b) Paola Sartoretti, CNRS Research Engineer & Gaia Collaboration Scientist, Observatoire de Paris – PSL- (English)
• June 13, 2022: Today, ESA’s Gaia mission releases its new treasure trove of data about our home galaxy. Astronomers describe strange ‘starquakes’, stellar DNA, asymmetric motions and other fascinating insights in this most detailed Milky Way survey to date. 39)
- Gaia is ESA’s mission to create the most accurate and complete multi-dimensional map of the Milky Way. This allows astronomers to reconstruct our home galaxy’s structure and past evolution over billions of years, and to better understand the lifecycle of stars and our place in the Universe (see video of Figure 20).
What’s New in Data Release 3?
- Gaia’s data release 3 contains new and improved details for almost two billion stars in our galaxy. The catalogue includes new information including chemical compositions, stellar temperatures, colours, masses, ages, and the speed at which stars move towards or away from us (radial velocity). Much of this information was revealed by the newly released spectroscopy data, a technique in which the starlight is split into its constituent colours (like a rainbow). The data also includes special subsets of stars, like those that change brightness over time.
- Also new in this data set is the largest catalogue yet of binary stars, thousands of Solar System objects such as asteroids and moons of planets, and millions of galaxies and quasars outside the Milky Way.

Radial velocity
- ESA’s Gaia data release 3 shows us the speed at which more than 30 million objects in the Milky Way (mostly stars) move towards or away from us. This is called ‘radial velocity’. We can now see how the objects move over a large portion of the Milky Way’s disc.
- The rotation of the disc, projected along the line-of-sight, is visible from the alternation of bright areas (moving away from us) and dark areas (moving toward us). Several objects whose radial velocity differs from that of their close environment are visible by contrast.
- The Large and Small Magellanic Clouds (LMC and SMC) appear as bright spots in the lower right corner of the image. The Sagittarius dwarf galaxy is visible as a faint quasi-vertical stripe below the Galactic Centre.
- Several globular clusters appear as tiny dots in the image, such as 47 Tucanae, the dark dot on the immediate left of the SMC.
Proper Motion
- This sky map shows the velocity field of the Milky Way for ~26 million stars. The colours show the radial velocities of stars along the line-of-sight. Blue shows the parts of the sky where the average motion of stars is towards us and red shows the regions where the average motion is away from us. The lines visible in the figure trace out the motion of stars projected on the sky (proper motion). These lines show how the direction of the speed of stars varies by galactic latitude and longitude. The Large and Small Magellanic Clouds (LMC and SMC) are not visible as only stars with well defined distances were selected to make this image.
Interstellar Dust
- Gaia not only maps the stars in our galaxy but tells us what is in between the stars. The space between stars is not empty but instead filled with dust and gas clouds, out of which stars are born.
- Through the precise measurements of the stars' positions and their dispersed light, Gaia allows us to map the absorption of the starlight by the interstellar medium. Those maps provide us with essential clues to the physical mechanisms of the formation of stars, galaxies, and the history of our home galaxy.
- This map shows the interstellar dust that fills the Milky Way. The dark regions in the centre of the Galactic plane in black are the regions with a lot of interstellar dust fading to the yellow as the amount of dust decreases. The dark blue regions above and below the Galactic plane are regions where there is little dust.
Chemical Map
- What stars are made of can tell us about their birthplace and their journey afterwards, and therefore about the history of the Milky Way. With today’s data release, Gaia is bringing us a chemical map of the galaxy.
- With Gaia, we see that some stars in our galaxy are made of primordial material, while others like our Sun are made of matter enriched by previous generations of stars. Stars that are closer to the centre and plane of our galaxy are richer in metals than stars at larger distances.
- This all-sky view shows a sample of the Milky Way stars in Gaia’s data release 3. The colour indicates the stellar metallicity. Redder stars are richer in metals.
Starquakes
- One of the most surprising discoveries coming out of the new data is that Gaia is able to detect starquakes – tiny motions on the surface of a star – that change the shapes of stars, something the observatory was not originally built for.
- Previously, Gaia already found radial oscillations that cause stars to swell and shrink periodically, while keeping their spherical shape. But Gaia has now also spotted other vibrations that are more like large-scale tsunamis. These nonradial oscillations change the global shape of a star and are therefore harder to detect.
- Gaia found strong nonradial starquakes in thousands of stars. Gaia also revealed such vibrations in stars that have seldomly been seen before. These stars should not have any quakes according to the current theory, while Gaia did detect them at their surface.
- “Starquakes teach us a lot about stars, notably their internal workings. Gaia is opening a goldmine for ‘asteroseismology' of massive stars,” says Conny Aerts of KU Leuven in Belgium, who is a member of the Gaia collaboration.
The DNA of stars
- What stars are made of can tell us about their birthplace and their journey afterwards, and therefore about the history of the Milky Way. With today’s data release, Gaia is revealing the largest chemical map of the galaxy coupled to 3D motions, from our solar neigbourhood to smaller galaxies surrounding ours.
- Some stars contain more ‘heavy metals’ than others. During the Big Bang, only light elements were formed (hydrogen and helium). All other heavier elements – called metals by astronomers – are built inside stars. When stars die, they release these metals into the gas and dust between the stars called the interstellar medium, out of which new stars form. Active star formation and death will lead to an environment that is richer in metals. Therefore, a star’s chemical composition is a bit like its DNA, giving us crucial information about its origin.
- With Gaia, we see that some stars in our galaxy are made of primordial material, while others like our Sun are made of matter enriched by previous generations of stars. Stars that are closer to the centre and plane of our galaxy are richer in metals than stars at larger distances. Gaia also identified stars that originally came from different galaxies than our own, based on their chemical composition.

Binary Stars, Asteroids, Quasars, and More
- Other papers that are published today reflect the breadth and depth of Gaia's discovery potential. A new binary star catalogue presents the mass and evolution of more than 800 thousand binary systems, while a new asteroid survey comprising 156 thousand rocky bodies is digging deeper into the origin of our Solar System. Gaia is also revealing information about 10 million variable stars, mysterious macro-molecules between stars, as well as quasars and galaxies beyond our own cosmic neighbourhood.


• May 13, 2022: ESA’s Gaia space telescope revolutionises our understanding of the Milky Way. It scans the sky to measure the position, movement, distance, and characteristics of billions of stars. 40)
- Gaia’s data release 3 will be made public on 13 June 2022: https://www.cosmos.esa.int/web/gaia/data-release-3
• March 23, 2022: Using data from ESA’s Gaia mission, astronomers have shown that a part of the Milky Way known as the ‘thick disc’ began forming 13 billion years ago, around 2 billion years earlier than expected, and just 0.8 billion years after the Big Bang. 41)
- This surprising result comes from an analysis performed by Maosheng Xiang and Hans-Walter Rix, from the Max-Planck Institute for Astronomy, Heidelberg, Germany. They took brightness and positional data from Gaia’s Early Data Release 3 (EDR3) dataset and combined it with measurements of the stars’ chemical compositions, as given by data from China’s Large Sky Area Multi-Object Fiber Spectroscopic Telescope (LAMOST) for roughly 250,000 stars to derive their ages.

- They chose to look at sub giant stars. In these stars, energy has stopped being generated in the star’s core and has moved into a shell around the core. The star itself is transforming into a red giant star. Because the sub giant phase is a relatively brief evolutionary phase in a star’s life, it permits its age to be determined with great accuracy, but it’s still a tricky calculation.
How Old are the Stars?
- The age of a star is one of the most difficult parameters to determine. It cannot be measured directly but must be inferred by comparing a star’s characteristics with computer models of stellar evolution. The compositional data helps with this. The Universe was born with almost exclusively hydrogen and helium. The other chemical elements, known collectively as metals to astronomers, are made inside stars, and exploded back into space at the end of a star’s life, where they can be incorporated into the next generation of stars. So, older stars have fewer metals and are said to have lower metallicity.
- The LAMOST data gives the metallicity. Together, the brightness and metallicity allow astronomers to extract the star’s age from the computer models. Before Gaia, astronomers were routinely working with uncertainties of 20-40 percent, which could result in the determined ages being imprecise by a billion years or more.
- The LAMOST data gives the metallicity. Together, the brightness and metallicity allow astronomers to extract the star’s age from the computer models. Before Gaia, astronomers were routinely working with uncertainties of 20-40 percent, which could result in the determined ages being imprecise by a billion years or more.
Milky Way Anatomy

- Our galaxy is made of different components. Broadly, these can be split into the halo and the disc. The halo is the spherical region surrounding the disc, and has traditionally been thought to be the oldest component of the galaxy. The disc is composed of two parts: the thin disc and the thick disc. The thin disc contains most of the stars that we see as the misty band of light in the night sky that we call the Milky Way. The thick disc is more than double the height of the thin disc but smaller in radius, containing only a few percent of the Milky Way’s stars in the solar neighbourhood.
- By identifying sub giant stars in these different regions, the researchers were able to build a timeline of the Milky Way’s formation – and that’s when they got a surprise.
Two Phases in Milky Way History
- The stellar ages clearly revealed that the formation of the Milky Way fell into two distinct phases. In the first phase, starting just 0.8 billion years after the Big Bang, the thick disc began forming stars. The inner parts of the halo may also have begun to come together at this stage, but the process rapidly accelerated to completion about two billion years later when a dwarf galaxy known as Gaia-Sausage-Enceladus merged with the Milky Way. It filled the halo with stars and, as clearly revealed by the new work, triggered the nascent thick disc to form the majority of its stars. The thin disc of stars which holds the Sun, was formed during the subsequent, second phase of the galaxy’s formation.
- The analysis also shows that after the star-forming burst triggered by the merger with Gaia-Sausage-Enceladus, the thick disc continued to form stars until the gas was used up at around 6 billion years after the Big Bang. During this time, the metallicity of the thick disk grew by more than a factor of 10. But remarkably, the researchers see a very tight stellar age—metallicity relation, which indicates that throughout that period, the gas forming the stars was well-mixed across the whole disk. This implies that the early Milky Way’s disk regions must have been formed from highly turbulent gas that effectively spread the metals far and wide.
A Timeline Thanks to Gaia
- The earlier formation age of the thick disc points to a different picture of our galaxy’s early history. “Since the discovery of the ancient merger with Gaia-Sausage-Enceladus, in 2018, astronomers have suspected that the Milky Way was already there before the halo formed, but we didn’t have a clear picture of what that Milky Way looked like. Our results provide exquisite details about that part of the Milky Way, such as its birthday, its star-formation rate and metal enrichment history. Putting together these discoveries using Gaia data is revolutionising our picture of when and how our galaxy was formed.” says Maosheng.
- And we may not yet be looking far enough into the Universe to see similar galactic discs forming. An age of 13 billion years corresponds to a redshift of 7, where redshift is a measure of how far away a celestial object is, and so how long its light has taken to cross space and reach us.
- New observations could come in the near future as the James Webb Space Telescope has been optimised to see the earliest Milky Way-like galaxies in the Universe. And on 13 June this year, Gaia will release its full third data release (Gaia DR3). This catalogue will include spectra and derived information like ages and metallicity, making studies like Maosheng’s even easier to conduct.
- “With each new analysis and data release, Gaia allows us to piece together the history of our galaxy in even more unprecedented detail. With the release of Gaia DR3 in June, astronomers will be able to enrich the story with even more details,” says Timo Prusti, Gaia Project Scientist for ESA.
- A time-resolved picture of our Milky Way’s early formation history” by Maosheng Xiang and Hans-Walter Rix is published in Nature. 42) doi 10.1038/s41586-022-04496-5
• March 16, 2022: On 18 February, the NASA/ESA/CSA James Webb Space Telescope was photographed by ESA’s Gaia observatory. 43)

- Both spacecraft are located in orbits around the Lagrange point 2 (L2), 1.5 million km from Earth in the direction away from the Sun. Gaia arrived there in 2014, and Webb in January 2022.
- On 18 February 2022, the two spacecraft were 1 million km apart, with an edge-on view of Gaia towards Webb’s huge sunshield. Very little reflected sunlight came Gaia’s way, and Webb therefore appears as a tiny, faint spec of light in Gaia’s two telescopes without any details visible.
Sky Mapper
- A few weeks before Webb’s arrival at L2, Gaia experts Uli Bastian of Heidelberg University (Germany) and Francois Mignard of Nice Observatory (France) realised that during Gaia’s continuous scanning of the entire sky, its new neighbour at L2 should occasionally cross Gaia’s fields of view.
- Gaia is not designed to take real pictures of celestial objects. Instead, it collects very precise measurements of their positions, motions, distances, and colours. However, one part of the instruments on board takes a sort of sky images. It is the ‘finder scope’ of Gaia, also called the sky mapper.
- Every six hours, Gaia’s sky mapper scans a narrow 360-degree strip around the entire celestial sphere. The successive strips are slightly tilted with respect to each other, so that every few months the entire sky is covered – touching everything that’s there and that’s bright enough to be seen by Gaia. Within seconds, these slices are automatically scrutinized for star images, the positions of which are then used to predict when and where those stars could be recorded in Gaia’s main scientific instruments. Then they are routinely deleted.
- But the computer can be manually requested to exceptionally keep a stretch of the image data. The sky mapper was originally planned for technical servicing purposes, but during the mission it has also found some scientific uses. Why not use it for a snapshot of Webb?

Got it!
- After Webb had reached its destination at L2, the Gaia scientists calculated when the first opportunity would arise for Gaia to spot Webb, which turned out to be 18 February 2022.
- After Gaia’s two telescopes had scanned the part of the sky where Webb would be visible, the raw data was downloaded to Earth. In the morning after, Francois sent an email to all people involved. The enthusiastic subject line of the email was "JWST: Got it!!"

- The astronomers had to wait a few more days for Juanma Martin-Fleitas, ESA’s Gaia calibration engineer, to identify Webb in the sky mapper images. "I've identified our target" was the message sent by him, with the images attached and the two tiny specks labelled as ‘Webb candidates’.
- After scrutinising these carefully, Uli replied: “Your ‘candidates’ can be safely renamed ‘Webb’”.
- Gaia now has a spacecraft friend at L2, and together they will uncover our home galaxy, and the Universe beyond.
• February 17, 2022: Our galaxy, the Milky Way, began forming around 12 billion years ago. Since then, it has been growing in both mass and size through a sequence of mergers with other galaxies. 44)
- Perhaps most exciting is that this process has not quite finished, and by using data from ESA’s Gaia spacecraft, astronomers can see it taking place. This in turn allows to reconstruct the history of our galaxy, revealing the ‘family tree’ of smaller galaxies that has helped make the Milky Way what it is today.

- The latest work on this subject comes from Khyati Malhan, a Humboldt Fellow at the Max-Planck-Institut für Astronomie, Heidelberg, Germany, and colleagues. Together, they have analysed data based on Gaia’s early third data release (EDR3) looking for the remains of smaller galaxies merging with our own. These can be found in the so-called halo of the Milky Way, which surrounds the disc of younger stars and central bulge of older stars that comprise the more luminous parts of the Milky Way.
- When a foreign galaxy falls into our own, great gravitational forces known as tidal forces pull it apart. If this process goes slowly, the stars from the merging galaxy will form a vast stellar stream that can be easily distinguished in the halo. If the process goes quickly, the merging galaxy’s stars will be more scattered throughout the halo and no clear signature will be visible.
- In total they studied 170 globular clusters, 41 stellar streams and 46 satellites of the Milky Way. Plotting them according to their energy and momentum revealed that 25 percent of these objects fall into six distinct groups. Each group is a merger taking place with the Milky Way. There was also a possible seventh merger in the data.
- Five had been previously identified on surveys of stars. They are known as Sagittarius, Cetus, Gaia-Sausage/Enceladus, LMS-1/Wukong, and Arjuna/Sequoia/I’itoi. But the sixth was a newly identified merger event. The team called it Pontus, meaning the sea. In Greek mythology, Pontus is the name of one of the first children of Gaia, the Greek goddess of the Earth.
- Based upon the way Pontus has been pulled apart by the Milky Way, Khyati and colleagues estimate that it probably fell into the Milky Way some eight to ten billion years ago. Four of the other five merger events likely also took place around this time as well. But the sixth event, Sagittarius, is more recent. It might have fallen into the Milky Way sometime in the last five to six billion years. As a result, the Milky Way has not yet been able to completely disrupt it.
- Piece by piece, astronomers are fitting together the merger history of the Galaxy, and Gaia data is proving invaluable.
- On 13 June 2022, the Gaia mission will issue its data release 3, which will provide even more detailed information about the Milky Way’s past, present, and future. 45)
• January 3, 2022: If it’s gigantic enough, a cold cloud of molecules can collapse and fragment under its own gravity to give birth to a litter of thousands of new stars: an open cluster. The stars’ mutual gravity is strong enough to hold the cluster together as it orbits its galactic host, but the attraction is too weak to keep cluster members from eventually straying, either on their own or as result of a dynamically disruptive event. 46)
- Galileo Galilei and other early telescope-wielding astronomers identified open clusters as improbable congregations of similar stars. Now clusters can be automatically cataloged by algorithms that trawl through astrometric data. In April 2021 Wilton Dias of the Federal University of Itajubá in Brazil and his collaborators published an updated catalog of 1743 open clusters based on an analysis of data gathered by the European Space Agency’s Gaia spacecraft. Andrés Piatti of the Interdisciplinary Institute of Basic Science in Mendoza, Argentina, and Khyati Malhan of Stockholm University in Sweden have now used that catalog set to look for pairs of clusters that are close together.
- Close clusters are rare. And when they do occur, they tend to be of similar ages, which suggests that they formed from the same giant molecular cloud. But the members of one pair that Piatti and Malhan found, IC 4665 (shown here) and Collinder 350, have ages that differ by more than 500 million years. What’s more, the clusters’ stellar populations overlap: IC 4665 and Collinder 350 appear to be merging.
- The disparate ages suggest that IC 4665 and Collinder 350 could have formed in different parts of the Milky Way. To see whether that was the case, Piatti and Malhan tracked the members of the two clusters back in time. They first noted the stars’ current positions in the six-dimensional phase space made up of the stars’ 3D positions and 3D velocities. For the source of gravity acting on the stars, they used two different continuous models of the distribution of gravitational mass in the galaxy. Both models include an extended disk, a central bulge, and a dark-matter halo. They differ in total mass.
- Piatti and Malhan integrated the stars’ equations of motion backward for 80 Myr. Although the two mass models gave slightly different answers, the result was the same: 60 Myr ago, IC 4665 and Collinder 350 were around 500 parsecs (1500 light-years) apart. That distance is more than twice as large as the diameters of the biggest molecular clouds. The two clusters had different parents.
- Whether IC 4665 and Collinder 350 will coalesce or separate after passing through each other remains to be determined. The question pertains to how an observed spread in the ages and chemical composition of a cluster are accounted for. If coalescence is a possibility, distinct episodes of star formation need not be invoked. 47)

• December 1, 2021: Astronomers at The University of Texas at Austin’s McDonald Observatory have discovered an unusually massive black hole at the heart of one of the Milky Way’s dwarf satellite galaxies, called Leo I. Almost as massive as the black hole in our own galaxy, the finding could redefine our understanding of how all galaxies — the building blocks of the universe — evolve. The work is published in a recent issue of The Astrophysical Journal. 48)
![Figure 38: McDonald Observatory astronomers have found that Leo I (inset), a tiny satellite galaxy of the Milky Way (main image), has a black hole nearly as massive as the Milky Way's. Leo I is 30 times smaller than the Milky Way. The result could signal changes in astronomers' understanding of galaxy evolution. Credit: ESA/Gaia/DPAC; SDSS (inset) McDonald Observatory astronomers have found that Leo I (inset), a tiny satellite galaxy of the Milky Way (main image), has a black hole nearly as massive as the Milky Way's. Leo I is 30 times smaller than the Milky Way. The result could signal changes in astronomers' understanding of galaxy evolution [image credit: ESA/Gaia/DPAC; SDSS (inset)]](https://www.eoportal.org/ftp/satellite-missions/g/Gaia_130622/Gaia_Auto2F.jpeg)
- The team decided to study Leo I because of its peculiarity. Unlike most dwarf galaxies orbiting the Milky Way, Leo I does not contain much dark matter. Researchers measured Leo I’s dark matter profile — that is, how the density of dark matter changes from the outer edges of the galaxy all the way into its center. They did this by measuring its gravitational pull on the stars: The faster the stars are moving, the more matter there is enclosed in their orbits. In particular, the team wanted to know whether dark matter density increases toward the galaxy’s center. They also wanted to know whether their profile measurement would match previous ones made using older telescope data combined with computer models.
- Led by recent UT Austin doctoral graduate María José Bustamante, the team includes UT astronomers Eva Noyola, Karl Gebhardt and Greg Zeimann, as well as colleagues from Germany’s Max Planck Institute for Extraterrestrial Physics (MPE). 49)
- For their observations, they used a unique instrument called VIRUS-W on McDonald Observatory’s 2.7-meter Harlan J. Smith Telescope.
- When the team fed their improved data and sophisticated models into a supercomputer at UT Austin’s Texas Advanced Computing Center, they got a startling result.
- “The models are screaming that you need a black hole at the center; you don’t really need a lot of dark matter,” Gebhardt said. “You have a very small galaxy that is falling into the Milky Way, and its black hole is about as massive as the Milky Way’s. The mass ratio is absolutely huge. The Milky Way is dominant; the Leo I black hole is almost comparable.” The result is unprecedented.
- The researchers said the result was different from the past studies of Leo I due to a combination of better data and the supercomputer simulations. The central, dense region of the galaxy was mostly unexplored in previous studies, which concentrated on the velocities of individual stars. The current study showed that for those few velocities that were taken in the past, there was a bias toward low velocities. This, in turn, decreased the inferred amount of matter enclosed within their orbits.
- The new data is concentrated in the central region and is unaffected by this bias. The amount of inferred matter enclosed within the stars’ orbits skyrocketed.
- The finding could shake up astronomers’ understanding of galaxy evolution, as “there is no explanation for this kind of black hole in dwarf spheroidal galaxies,” Bustamante said.
- The result is all the more important as astronomers have used galaxies such as Leo I, called “dwarf spheroidal galaxies,” for 20 years to understand how dark matter is distributed within galaxies, Gebhardt added. This new type of black hole merger also gives gravitational wave observatories a new signal to search for.
- “If the mass of Leo I’s black hole is high, that may explain how black holes grow in massive galaxies,” Gebhardt said. That’s because over time, as small galaxies like Leo I fall into larger galaxies, the smaller galaxy’s black hole merges with that of the larger galaxy, increasing its mass.
- Built by a team at MPE in Germany, VIRUS-W is the only instrument in the world now that can do this type of dark matter profile study. Noyola pointed out that many southern hemisphere dwarf galaxies are good targets for it, but no southern hemisphere telescope is equipped for it. However, the Giant Magellan Telescope (GMT) now under construction in Chile was, in part, designed for this type of work. UT Austin is a founding partner of the GMT.
• November 24, 2021: Data from ESA’s Gaia mission is re-writing the history of our galaxy, the Milky Way. What had traditionally been thought of as satellite galaxies to the Milky Way are now revealed to be mostly newcomers to our galactic environment. 50)
- A dwarf galaxy is a collection of between thousand and several billion stars. For decades it has been widely believed that the dwarf galaxies that surround the Milky Way are satellites, meaning that they are caught in orbit around our galaxy, and have been our constant companions for many billions of years. Now the motions of these dwarf galaxies have been computed with unprecedented precision thanks to data from Gaia’s early third data release and the results are surprising.
- François Hammer, Observatoire de Paris - Université Paris Sciences et Lettres, France, and colleagues from across Europe and China, used the Gaia data to calculate the movements of 40 dwarf galaxies around the Milky Way. They did this by computing a set of quantities known as the three-dimensional velocities for each galaxy, and then using those to calculate the galaxy’s orbital energy and the angular (rotational) momentum.

- They found that these galaxies are moving much faster than the giant stars and star clusters that are known to be orbiting the Milky Way. So fast, that they couldn’t be in orbit yet around the Milky Way, where interactions with our galaxy and its contents would have sapped their orbital energy and angular momentum.
- Our galaxy has cannibalized a number of dwarf galaxies in its past. For example, 8-10 billion years ago, a dwarf galaxy called Gaia-Enceladus was absorbed by the Milky Way. Its stars can be identified in Gaia data because of the eccentric orbits and range of energies they possess.
- More recently, 4-5 billion years ago, the Sagittarius dwarf galaxy was captured by the Milky Way and is currently in the process of being pulled to pieces and assimilated. The energy of its stars is higher than those of Gaia-Enceladus, indicating the shorter time that they have been subject to the Milky Way’s influence.
- In the case of the dwarf galaxies in the new study, which represents the majority of the dwarf galaxies around the Milky Way, their energies are higher still. This strongly suggests that they have only arrived in our vicinity in the last few billion years.
- The discovery mirrors one made about the Large Magellanic Cloud (LMC), a larger dwarf galaxy so close to the Milky Way that it is visible as a smudge of light in the night sky from the southern hemisphere. The LMC was also thought to be a satellite galaxy of the Milky Way until the 2000s, when astronomers measured its velocity and found that it was travelling too fast to be gravitationally bound. Instead of a companion, LMC is visiting for the first time. Now we know that the same is true for most of the dwarf galaxies too.
- So will these newcomers settle into orbit or simply pass us by? “Some of them will be captured by the Milky Way and will become satellites,” says François.
- But saying exactly which ones is difficult because it depends on the exact mass of the Milky Way, and that is a quantity that is difficult for astronomers to calculate with any real accuracy. Estimates vary by a factor of two.
- The discovery of the dwarf galaxy energies is significant because it forces us to re-evaluate the nature of the dwarf galaxies themselves.
- As a dwarf galaxy orbits, the Milky Way’s gravitational pull will try to wrench it apart. In physics this is known as a tidal force. “The Milky Way is a big galaxy, so its tidal force is simply gigantic and it's very easy to destroy a dwarf galaxy after maybe one or two passages,” says François.
- In other words, becoming a companion to the Milky Way is a death sentence for dwarf galaxies. The only thing that could resist our galaxy’s destructive grip is if the dwarf had a significant quantity of dark matter. Dark matter is the mysterious substance that astronomers think exists in the universe to provide the extra gravity to hold individual galaxies together.
- And so, in the traditional view that the Milky Way’s dwarfs were satellite galaxies that had been in orbit for many billions of years, it was assumed that they must be dominated by dark matter to balance the Milky Way’s tidal force and keep them intact. The fact that Gaia has revealed that most of the dwarf galaxies are circling the Milky Way for the first time means that they do not necessarily need to include any dark matter at all, and we must re-assess whether these systems are in balance or rather in the process of destruction.
- “Thanks in large part to Gaia, it is now obvious that the history of the Milky Way is far more storied than astronomers had previously understood. By investigating these tantalizing clues, we hope to further tease out the fascinating chapters in our galaxy’s past,” says Timo Prusti, Gaia Project Scientist, ESA (publication in The Astrophysical Journal). 51)
• June 7, 2021: It's hard to see more than a handful of stars from Princeton University, because the lights from New York City, Princeton and Philadelphia prevent our sky from ever getting pitch black, but stargazers who get into more rural areas can see hundreds of naked-eye stars—and a few smudgy objects, too. 52)

- The biggest smudge is the Milky Way itself, the billions of stars that make up our spiral galaxy, which we see edge-on. The smaller smudges don't mean that you need glasses, but that you're seeing tightly packed groups of stars. One of the best-known of these "clouds" or "clusters"—groups of stars that travel together—is the Pleiades, also known as the Seven Sisters. Clusters are stellar nurseries where thousands of stars are born from clouds of gas and dust and then disperse across the Milky Way.
- For centuries, scientists have speculated about whether these clusters always form tight clumps like the Pleiades, spread over only a few dozen lightyears.
- "We call them 'open clusters'—the 'open' part refers to the expectation that these things formed in a much denser group that then dispersed," said Luke Bouma, a graduate student in astrophysical sciences at Princeton and the lead author on an upcoming paper published by the American Astronomical Society. "But we never thought we'd be able to find the stars that were lost."
- Then, two years ago, a machine-learning algorithm using data from the Gaia satellite identified that many far-flung stars are moving at the same speed and direction and could therefore be part of the same open cluster—but as more of a stream or a string than a clump.
- Now, a team of astrophysicists led by Bouma can confirm that one of these streams of stars, NGC 2516, also known as the Southern Beehive, extends at least 1,600 light-years—500 parsecs—from tip to tip. To an Earth-based stargazer, that would look as big as 40 full moons, side by side, stretching across the sky.
- "Gaia data let us trace the process of star cluster formation and dissolution in unprecedented detail—but to complete the picture, we need independently estimated ages," said Lynne Hillenbrand, a 1989 Princeton alumna and a professor of astronomy at Caltech, who was not involved in this research. "Bouma's paper brings together several different methods to consistently age-date stars at both the core and the outer reaches of this cluster."
- "In retrospect, the existence of this large stellar stream is not too surprising," said Bouma, who recently won the prestigious 51 Pegasus b Fellowship. One interpretation could be that a cluster starts as a tight clump that expands through time to form "tidal tails" stretching in front of it and behind it, as it moves through the Milky Way.
- "The broader implication is that there are bound to be other enormous open clusters like this," he said. "The visible part of the cluster, where we can easily see the stars close together, may be only a small part of a much, much larger stream."
- "I have seen the Southern Beehive many times through a pair of binoculars under the dark skies of Chile," said Gaspar Bakos, a professor of astrophysical sciences and the director of Princeton's program in planets and life, who was a co-author on the paper. "The cluster nicely fits the view of the binoculars, because its apparent size in the sky is something like the tip of my thumb at arm's length. It is curious to know, thanks to Luke's research, that the cluster actually spans an area as big as my entire palm held toward the sky."
- Bouma and his colleagues used data from the Transiting Exoplanet Survey Satellite (TESS) to precisely measure the rotation rates of stars that the Gaia study had assigned to NGC 2516. The researchers demonstrated that many stars with similar masses are all spinning at (or very near) the same rate, confirming that they were born in the same stellar nursery.
- Bouma has spent years developing the tools to measure a star's rotation so that he can calculate its age, a technique called gyrochronology (from the Greek words for "spin" and "time"). Our sun, which at the age of 4.6 billion years old is in its sedate middle age, rotates once every 27 days. The stars Bouma measured in NGC 2516 are rotating 10 times faster than our sun, because they are so much younger. Those stars are barely out of their infancy, only about 150 million years old.
- "In addition to expanding our knowledge of this and other star clusters, Luke has given us an expanded list of young stars that we can search for planets," said Joshua Winn, Bouma's adviser and co-author and a professor of astrophysical sciences. "Finding planets around young stars will help us understand how planetary systems form and change with time."
- "What's so surprising about this work - what's so exciting - is that we confirmed that Gaia, because it really precisely measures the positions and the motions of stars, can find these 'needles in the haystack' of the Milky Way," Bouma said. "Gaia can identify all the stars that are moving in the same direction, at the same rate. And we don't have to just trust the machine learning algorithm saying that they're related - we can verify it with TESS data, using our gyrochronological technique."
- This open cluster also has an intriguing connection with Greek mythology, Bouma said. "In the southern night sky, NGC 2516 is near a constellation called the Argo Navis, which was the boat sailed by Jason and the Argonauts to obtain the golden fleece." He added with a smile: "Jason and the Argonauts are sailing on a stream of stars made by the open cluster NGC 2516." 53)
• April 21, 2021: Astronomers using data from NASA and ESA (European Space Agency) telescopes have released a new all-sky map of the outermost region of our galaxy. Known as the galactic halo, this area lies outside the swirling spiral arms that form the Milky Way’s recognizable central disk and is sparsely populated with stars. Though the halo may appear mostly empty, it is also predicted to contain a massive reservoir of dark matter, a mysterious and invisible substance thought to make up the bulk of all the mass in the universe. 54)

- Astronomers using data from NASA and ESA (European Space Agency) telescopes have released a new all-sky map of the outermost region of our galaxy. Known as the galactic halo, this area lies outside the swirling spiral arms that form the Milky Way’s recognizable central disk and is sparsely populated with stars. Though the halo may appear mostly empty, it is also predicted to contain a massive reservoir of dark matter, a mysterious and invisible substance thought to make up the bulk of all the mass in the universe.
- The data for the new map comes from ESA’s Gaia mission and NASA’s NEOWISE (Near Earth Object Wide Field Infrared Survey Explorer) mission, which operated from 2009 to 2013 under the moniker WISE. The study makes use of data collected by the spacecraft between 2009 and 2018.
- The new map reveals how a small galaxy called the Large Magellanic Cloud (LMC) – so named because it is the larger of two dwarf galaxies orbiting the Milky Way – has sailed through the Milky Way’s galactic halo like a ship through water, its gravity creating a wake in the stars behind it. The LMC is located about 160,000 light-years from Earth and is less than one-quarter the mass of the Milky Way.
- Though the inner portions of the halo have been mapped with a high level of accuracy, this is the first map to provide a similar picture of the halo’s outer regions, where the wake is found – about 200,000 light-years to 325,000 light-years from the galactic center. Previous studies have hinted at the wake’s existence, but the all-sky map confirms its presence and offers a detailed view of its shape, size, and location.
- This disturbance in the halo also provides astronomers with an opportunity to study something they can’t observe directly: dark matter. While it doesn’t emit, reflect, or absorb light, the gravitational influence of dark matter has been observed across the universe. It is thought to create a scaffolding on which galaxies are built, such that without it, galaxies would fly apart as they spin. Dark matter is estimated to be five times more common in the universe than all the matter that emits and/or interacts with light, from stars to planets to gas clouds.
- Although there are multiple theories about the nature of dark matter, all of them indicate that it should be present in the Milky Way’s halo. If that’s the case, then as the LMC sails through this region, it should leave a wake in the dark matter as well. The wake observed in the new star map is thought to be the outline of this dark matter wake; the stars are like leaves on the surface of this invisible ocean, their position shifting with the dark matter.
- The interaction between the dark matter and the Large Magellanic Cloud has big implications for our galaxy. As the LMC orbits the Milky Way, the dark matter’s gravity drags on the LMC and slows it down. This will cause the dwarf galaxy’s orbit to get smaller and smaller, until the galaxy finally collides with the Milky Way in about 2 billion years. These types of mergers might be a key driver in the growth of massive galaxies across the universe. In fact, astronomers think the Milky Way merged with another small galaxy about 10 billion years ago.
- “This robbing of a smaller galaxy’s energy is not only why the LMC is merging with the Milky Way, but also why all galaxy mergers happen,” said Rohan Naidu, a doctoral student in astronomy at Harvard University and a co-author of the new paper. “The wake in our map is a really neat confirmation that our basic picture for how galaxies merge is on point!”55)
A Rare Opportunity
- The authors of the paper also think the new map – along with additional data and theoretical analyses – may provide a test for different theories about the nature of dark matter, such as whether it consists of particles, like regular matter, and what the properties of those particles are.
- “You can imagine that the wake behind a boat will be different if the boat is sailing through water or through honey,” said Charlie Conroy, a professor at Harvard University and an astronomer at the Center for Astrophysics | Harvard & Smithsonian, who coauthored the study. “In this case, the properties of the wake are determined by which dark matter theory we apply.”
- Conroy led the team that mapped the positions of over 1,300 stars in the halo. The challenge arose in trying to measure the exact distance from Earth to a large portion of those stars: It’s often impossible to figure out whether a star is faint and close by or bright and far away. The team used data from ESA’s Gaia mission, which provides the location of many stars in the sky but cannot measure distances to the stars in the Milky Way’s outer regions.
- After identifying stars most likely located in the halo (because they were not obviously inside our galaxy or the LMC), the team looked for stars belonging to a class of giant stars with a specific light “signature” detectable by NEOWISE. Knowing the basic properties of the selected stars enabled the team to figure out their distance from Earth and create the new map. It charts a region starting about 200,000 light-years from the Milky Way’s center, or about where the LMC’s wake was predicted to begin, and extends about 125,000 light-years beyond that.
- Conroy and his colleagues were inspired to hunt for LMC’s wake after learning about a team of astrophysicists at the University of Arizona in Tucson that makes computer models predicting what dark matter in the galactic halo should look like. The two groups worked together on the new study.
- One model by the Arizona team, included in the new study, predicted the general structure and specific location of the star wake revealed in the new map. Once the data had confirmed that the model was correct, the team could confirm what other investigations have also hinted at: that the LMC is likely on its first orbit around the Milky Way. If the smaller galaxy had already made multiple orbits, the shape and location of the wake would be significantly different from what has been observed. Astronomers think the LMC formed in the same environment as the Milky Way and another nearby galaxy, M31, and that it is close to completing a long first orbit around our galaxy (about 13 billion years). Its next orbit will be much shorter due to its interaction with the Milky Way.
- “Confirming our theoretical prediction with observational data tells us that our understanding of the interaction between these two galaxies, including the dark matter, is on the right track,” said University of Arizona doctoral student in astronomy Nicolás Garavito-Camargo, who led work on the model used in the paper.
- The new map also provides astronomers with a rare opportunity to test the properties of the dark matter (the notional water or honey) in our own galaxy. In the new study, Garavito-Camargo and colleagues used a popular dark matter theory called cold dark matter that fits the observed star map relatively well. Now the University of Arizona team is running simulations that use different dark matter theories to see which one best matches the wake observed in the stars.
- “It’s a really special set of circumstances that came together to create this scenario that lets us test our dark matter theories,” said Gurtina Besla, a co-author of the study and an associate professor at the University of Arizona. “But we can only realize that test with the combination of this new map and the dark matter simulations that we built.”
- Launched in 2009, the WISE spacecraft was placed into hibernation in 2011 after completing its primary mission. In September 2013, NASA reactivated the spacecraft with the primary goal of scanning for near-Earth objects, or NEOs, and the mission and spacecraft were renamed NEOWISE. NASA’s Jet Propulsion Laboratory in Southern California managed and operated WISE for NASA’s Science Mission Directorate. The mission was selected competitively under NASA’s Explorers Program managed by the agency’s Goddard Space Flight Center in Greenbelt, Maryland. NEOWISE is a project of JPL, a division of Caltech, and the University of Arizona, supported by NASA’s Planetary Defense Coordination Office.
• March 24, 2021: Data from ESA’s Gaia star mapping satellite have revealed tantalizing evidence that the nearest star cluster to the Sun is being disrupted by the gravitational influence of a massive but unseen structure in our galaxy. 56)

- If true, this might provide evidence for a suspected population of ‘dark matter sub-halos’. These invisible clouds of particles are thought to be relics from the formation of the Milky Way, and are now spread across the galaxy, making up an invisible substructure that exerts a noticeable gravitational influence on anything that drifts too close.
- ESA Research Fellow Tereza Jerabkova and colleagues from ESA and the European Southern Observatory made the discovery while studying the way a nearby star cluster is merging into the general background of stars in our galaxy. This discovery was based on Gaia’s Early third Data Release (EDR3) and data from the second release.

- The team chose the Hyades as their target because it is the nearest star cluster to the Sun. It is located just over 153 light years away, and is easily visible to skywatchers in both northern and southern hemispheres as a conspicuous ‘V’ shape of bright stars that marks the head of the bull in the constellation of Taurus. Beyond the easily visible bright stars, telescopes reveal a hundred or so fainter ones contained in a spherical region of space, roughly 60 light years across.
- A star cluster will naturally lose stars because as those stars move within the cluster they tug at each other gravitationally. This constant tugging slightly changes the stars’ velocities, moving some to the edges of the cluster. From there, the stars can be swept out by the gravitational pull of the galaxy, forming two long tails.
- One tail trails the star cluster, the other pulls out ahead of it. They are known as tidal tails, and have been widely studied in colliding galaxies but no one had ever seen them from a nearby open star cluster, until very recently.
- The key to detecting tidal tails is spotting which stars in the sky are moving in a similar way to the star cluster. Gaia makes this easy because it is precisely measuring the distance and movement of more than a billion stars in our galaxy. “These are the two most important quantities that we need to search for tidal tails from star clusters in the Milky Way,” says Tereza.
- Previous attempts by other teams had met with only limited success because the researchers had only looked for stars that closely matched the movement of the star cluster. This excluded members that left earlier in its 600–700 million year history and so are now travelling on different orbits.
- To understand the range of orbits to look for, Tereza constructed a computer model that would simulate the various perturbations that escaping stars in the cluster might feel during their hundreds of millions of years in space. It was after running this code, and then comparing the simulations to the real data that the true extend of the Hyades tidal tails were revealed. Tereza and colleagues found thousands of former members in the Gaia data. These stars now stretch for thousands of light years across the galaxy in two enormous tidal tails.
- But the real surprise was that the trailing tidal tail seemed to be missing stars. This indicates that something much more brutal is taking place than the star cluster gently ‘dissolving’.
- Running the simulations again, Tereza showed that the data could be reproduced if that tail had collided with a cloud of matter containing about 10 million solar masses. “There must have been a close interaction with this really massive clump, and the Hyades just got smashed,” she says.
- But what could that clump be? There are no observations of a gas cloud or star cluster that massive nearby. If no visible structure is detected even in future targeted searches, Tereza suggests that object could be a dark matter sub-halo. These are naturally occurring clumps of dark matter that are thought to help shape the galaxy during its formation. This new work shows how Gaia is helping astronomers map out this invisible dark matter framework of the galaxy.
- “With Gaia, the way we see the Milky Way has completely changed. And with these discoveries, we will be able to map the Milky Way’s sub-structures much better than ever before,” says Tereza. And having proved the technique with the Hyades, Tereza and colleagues are now extending the work by looking for tidal tails from other, more distant star clusters. 57)
• December 03, 2020: The motion of stars in the outskirts of our galaxy hints at significant changes in the history of the Milky Way. This and other equally fascinating results come from a set of papers that demonstrate the quality of ESA’s Gaia Early third Data Release (EDR3), which is made public today. 58)

- Gaia EDR3 includes:
a) 1,811,709,771 sources with positions to provide the best ever sky map
b) 1,467,744,818 sources with parallax and proper motion to reveal their distances and motions
c) 1,806,254,432 sources with the measurement of their brightness in white light
d) 1,542,033,472 sources with the brightness of the objects in blue light
e) 1,554,997,939 sources with the brightness of the objects in red light (a comparison of the blue and the red light provides information of the temperature of the object)
f) 1,614,173 extragalactic sources to provide a reference frame for measuring ‘absolute’ positions and motions.

What’s New in EDR3?
- Gaia EDR3 contains detailed information on more than 1.8 billion sources, detected by the Gaia spacecraft. This represents an increase of more than 100 million sources over the previous data release (Gaia DR2), which was made public in April 2018. Gaia EDR3 also contains color information for around 1.5 billion sources, an increase of about 200 million sources over Gaia DR2. As well as including more sources, the general accuracy and precision of the measurements has also improved.
- “The new Gaia data promise to be a treasure trove for astronomers,” says Jos de Bruijne, ESA’s Gaia Deputy Project Scientist.

![Figure 50: Gaia’s view of the Milky Way’s neighboring galaxies. The Large and Small Magellanic Clouds (LMC and SMC, respectively) are two dwarf galaxies that orbit the Milky Way. This image shows the stellar density of the satellite galaxies as seen by Gaia in its Early Data Release 3, which was made public on 3 December 2020. It is composed of red, green and blue layers, which trace mostly the older, intermediate age, and younger stars respectively. Astronomers place stars into categories that are often named for their color and appearance. - In this image, the red layer contains evolved stars that compose the Red Giant Branch and Red Clump stars. The green layer contains Main Sequence stars of mixed ages of up to two billion years. The blue layer contains stars younger than 400 million years, Asymptotic Giant Branch stars, and RR-Lyrae and classical Cepheid variable stars. - The brightnesses used in this image are based on a logarithmic scale to enhance low surface density regions in the galaxies, for example the outer spiral arm in the LMC visible in the upper left.- The density of younger stars has been artificially enhanced with respect to the other evolutionary phases to make them more clearly visible. This shows that younger stars mostly trace the inner spiral structure of the LMC, and the ‘bridge’ of stars between the two galaxies. Finally, intermediate age and older stars trace the LMC bar, spiral arms, and outer halo, as well as the SMC outer halo [image credit: ESA/Gaia/DPAC; CC BY-SA 3.0 IGO. Acknowledgement: L. Chemin; X. Luri et al (2020)]](https://www.eoportal.org/ftp/satellite-missions/g/Gaia_130622/Gaia_Auto26.jpeg)
To the Galactic Anticenter
- The new Gaia data have allowed astronomers to trace the various populations of older and younger stars out towards the very edge of our galaxy – the galactic anticenter. Computer models predicted that the disc of the Milky Way will grow larger with time as new stars are born. The new data allow us to see the relics of the 10 billion-year-old ancient disc and so determine its smaller extent compared to the Milky Way’s current disc size.
- The new data from these outer regions also strengthen the evidence for another major event in the more recent past of the galaxy.
- The data show that in the outer regions of the disc there is a component of slow-moving stars above the plane of our galaxy that are heading downwards towards the plane, and a component of fast-moving stars below the plane that are moving upwards. This extraordinary pattern had not been anticipated before. It could be the result of the near-collision between the Milky Way and the Sagittarius dwarf galaxy that took place in our galaxy’s more recent past.
- The Sagittarius dwarf galaxy contains a few tens of millions of stars and is currently in the process of being cannibalized by the Milky Way. Its last close pass to our galaxy was not a direct hit, but this would have been enough so that its gravity perturbed some stars in our galaxy like a stone dropping into water.
- Using Gaia DR2, members of DPAC had already found a subtle ripple in the movement of millions of stars that suggested the effects of the encounter with Sagittarius sometime between 300 and 900 million years ago. Now, using Gaia EDR3, they have uncovered more evidence that points to its strong effects on our galaxy’s disc of stars.
- “The patterns of movement in the disc stars are different to what we used to believe,” says Teresa Antoja, University of Barcelona, Spain, who worked on this analysis with DPAC colleagues. Although the role of the Sagittarius dwarf galaxy is still debated in some quarters, Teresa says, “It could be a good candidate for all these disturbances, as some simulations from other authors show.”

Measuring the Solar System’s Orbit
- The history of the galaxy is not the only result from the Gaia EDR3 demonstration papers. DPAC members across Europe have performed other work to demonstrate the extreme fidelity of the data and the unique potential for unlimited scientific discovery.
- In one paper, Gaia has allowed scientists to measure the acceleration of the Solar System with respect to the rest frame of the Universe. Using the observed motions of extremely distant galaxies, the velocity of the Solar System has been measured to change by 0.23 nm/s2 . Because of this tiny acceleration, the trajectory of the Solar System is deflected by the diameter of an atom every second, and in a year this adds up to around 115 km. The acceleration measured by Gaia shows a good agreement with the theoretical expectations and provides the first measurement of the curvature of the Solar System’s orbit around the galaxy in the history of optical astronomy.
A New Stellar Census
- Gaia EDR3 has also allowed a new census of stars in the solar neighborhood to be obtained. The Gaia Catalogue of Nearby Stars contains 331 312 objects, which is estimated to be 92 percent of the stars within 100 parsecs (326 light years) of the Sun. The previous census of the solar neighborhood, called the Gliese Catalogue of Nearby stars, was carried out in 1957. It possessed just 915 objects initially, but was updated in 1991 to 3803 celestial objects. It was also limited to a distance of 82 light years: Gaia’s census reaches four times farther and contains 100 times more stars. It also provides location, motion, and brightness measurements that are orders of magnitude more precise than the old data.
Beyond the Milky Way
- A fourth demonstration paper analyzed the Magellanic Clouds: two galaxies that orbit the Milky Way. Having measured the movement of the Large Magellanic Cloud’s stars to greater precision than before, Gaia EDR3 clearly shows that the galaxy has a spiral structure. The data also resolve a stream of stars that is being pulled out of the Small Magellanic Cloud, and hints at previously unseen structures in the outskirts of both galaxies.
- At 12:00 CET on 3 December, the data produced by the many scientists and engineers of the Gaia DPAC Consortium become public for anyone to look at and learn from. This is the first of a two-part release; the full Data Release 3 is planned for 2022.
![Figure 55: Bridge of stars. Data from Gaia’s Early Data Release 3 shows how stars are being pulled from the Small Magellanic Cloud, and heading towards the adjacent Large Magellanic Cloud, forming a stellar bridge through space [image credit: ESA/Gaia/DPAC; CC BY-SA 3.0 IGO. Acknowledgements: S. Jordan, T. Sagristà, X. Luri et al (2020)]](https://www.eoportal.org/ftp/satellite-missions/g/Gaia_130622/Gaia_Auto24.jpeg)
- “Gaia EDR3 is the result of a huge effort from everyone involved in the Gaia mission. It’s an extraordinarily rich data set, and I look forward to the many discoveries that astronomers from around the world will make with this resource,” says Timo Prusti, ESA’s Gaia Project Scientist. “And we’re not done yet; more great data will follow as Gaia continues to make measurements from orbit.”

• October 15, 2020: Star clusters have been part of the Imaginarium of human civilization for millennia, as shown through their countless representations in arts and sciences across cultures and continents. The closest and brightest star clusters to Earth, like the Pleiades, are readily visible to the naked eye and are prominent members of our night sky, where they appear as tight concentrations of stars. A research team around astronomer Stefan Meingast at the University of Vienna has now revealed the existence of massive stellar halos, termed coronae, surrounding local star clusters. The paper will be published in "Astronomy & Astrophysics". 60) 61)
- "Clusters form big families of stars that can stay together for large parts of their lifetime. Today, we know of roughly a few thousand star clusters in the Milky Way, but we only recognize them because of their prominent appearance as rich and tight groups of stars. Given enough time, stars tend to leave their cradle and find themselves surrounded by countless strangers, thereby becoming indistinguishable from their neighbors and hard to identify" says Stefan Meingast, lead author of the paper published in "Astronomy & Astrophysics". "Our Sun is thought to have formed in a star cluster but has left its siblings behind a long time ago" he adds.
- Thanks to the ESA Gaia spacecraft’s precise measurements, astronomers at the University of Vienna have now discovered that what we call a star cluster is only the tip of the iceberg of a much larger and often distinctly elongated distribution of stars.
- "Our measurements reveal the vast numbers of sibling stars surrounding the well-known cores of the star clusters for the first time. It appears that star clusters are enclosed in rich halos, or coronae, more than 10 times as large as the original cluster, reaching far beyond our previous guesses. The tight groups of stars we see in the night sky are just a part of a much larger entity" says Alena Rottensteiner, co-author and master student at the University of Vienna. "There is plenty of work ahead revising what we thought were basic properties of star clusters, and trying to understand the origin of the newfound coronae."
- To find the lost star siblings, the research team developed a new method that uses machine learning to trace groups of stars which were born together and move jointly across the sky. The team analyzed 10 star clusters and identified thousands of siblings far away from the center of the compact clusters, yet clearly belonging to the same family. An explanation for the origin of these coronae remains uncertain, yet the team is confident that their findings will redefine star clusters and aid our understanding of their history and evolution across cosmic time.
- "The star clusters we investigated were thought to be well-known prototypes, studied for more than a century, yet it seems we have to start thinking bigger. Our discovery will have important implications for our understanding of how the Milky Way was built, cluster by cluster, but also implications for the survival rate of proto-planets far from the sterilizing radiation of massive stars in the centers of clusters", says João Alves, Professor of Stellar Astrophysics at the University of Vienna and a co-author of the paper. "Dense star clusters with their massive but less dense coronae might not be a bad place to raise infant planets after all."
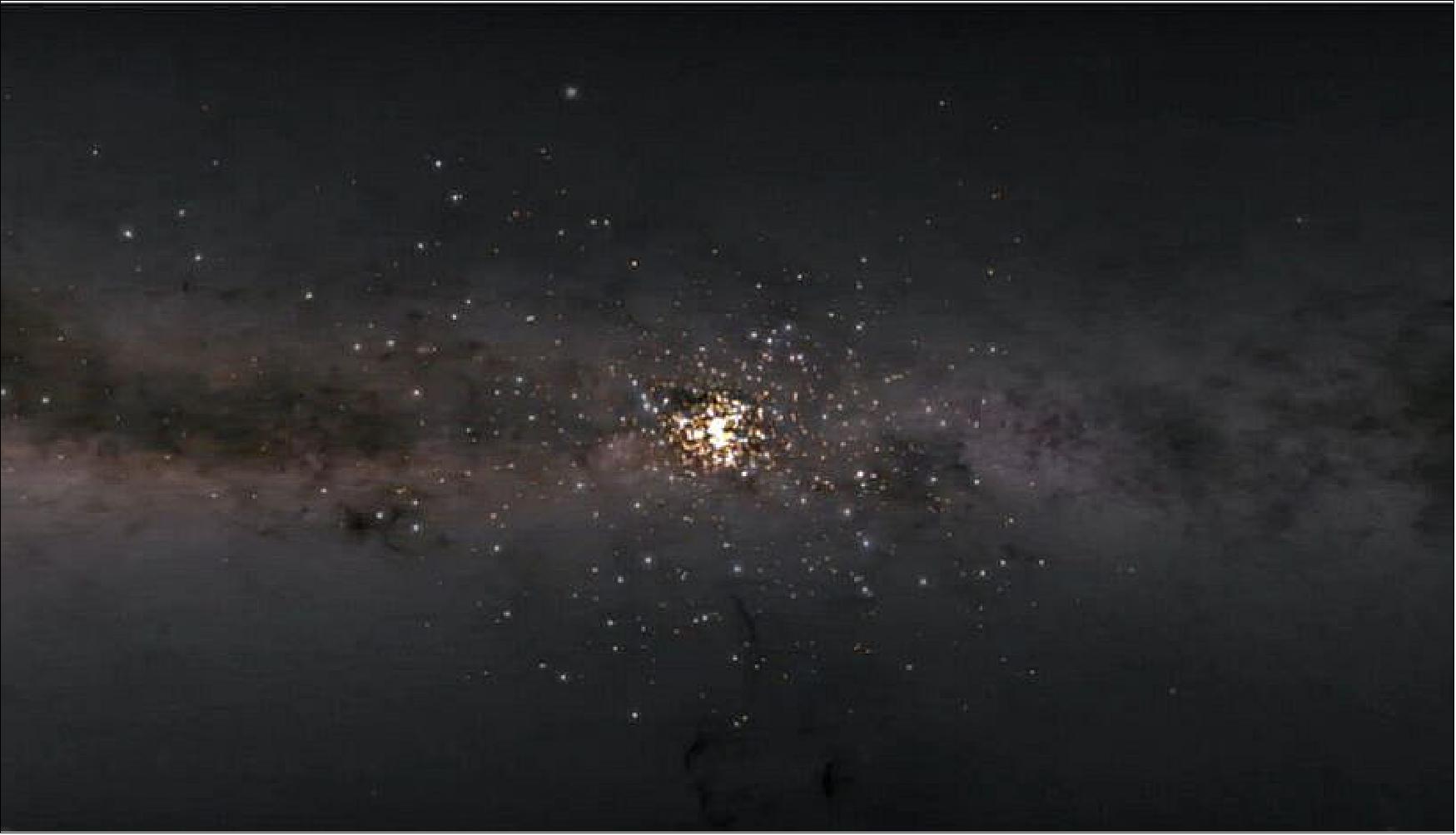
• July 14, 2020: On this page a description is given of the expected contents of Gaia's Early Data Release 3. The Gaia EDR3 catalogue will be based on 34 months of data collection, and is expected to contain about 1.8 billion stars. Gaia EDR3 is on track for a release late 2020. A more exact date will be announced later. 62)
• July 1, 2020: ESA’s Gaia space observatory is an ambitious mission to construct a three-dimensional map of our galaxy by making high-precision measurements of over one billion stars. However, on its journey to map distant suns, Gaia is revolutionizing a field much closer to home. By accurately mapping the stars, it is helping researchers track down lost asteroids. 63)
Using Stars to Spot Asteroids
- Gaia charts the galaxy by repeatedly scanning the entire sky. Over the course of its planned mission, it observed each of its more than one billion target stars around 70 times to study how their position and brightness change over time.

- The stars are so far from Earth that their movements between images are very small, hence why Gaia has to measure their positions so accurately to even notice a difference. However, sometimes Gaia spots faint light sources that move considerably from one image of a certain region of the sky to the next, or are even only spotted in a single image before disappearing.
- To move across Gaia’s field of view so quickly, these objects must be located much closer to Earth.
- By checking the positions of these objects against the catalogues of known Solar System bodies, many of these objects turn out to be known asteroids. Some, however, are identified as potentially new detections and are then followed up by the astronomy community through the Gaia Follow-Up Network for Solar System Objects. Through this process, Gaia has successfully discovered new asteroids.
Lost and Found
- These direct asteroid observations are important for solar system scientists. However, Gaia’s highly accurate measurements of the positions of stars provide an even more impactful, but indirect, benefit for asteroid tracking.
- “When we observe an asteroid, we look at its motion relative to the background stars to determine its trajectory and predict where it will be in the future,” says Marco Micheli from ESA’s Near-Earth Object Coordination Centre. “This means that the more accurately we know the positions of the stars, the more reliably we can determine the orbit of an asteroid passing in front of them.”

- In collaboration with the European Southern Observatory (ESO), Marco’s team took part in an observation campaign targeting 2012 TC4, a small asteroid that was due to pass by the Earth. Unfortunately, since the asteroid was first spotted in 2012, it had become fainter and fainter as it receded form Earth, eventually becoming unobservable. Where it would appear in the sky at the time of the upcoming campaign was not well known.
- “The possible region of the sky where the asteroid might appear was larger than the area that the telescope could observe at one time,” says Marco. “So we had to find a way to improve our prediction of where the asteroid would be.”
- “I looked back at the initial observations from 2012. Gaia had since made more accurate measurements of the positions of some of the stars in the background of the images, and I used these to update our understanding of the asteroid’s trajectory and predict where it would appear.”
- “We pointed the telescope towards the predicted area of the sky using the data from Gaia and we found the asteroid on our first attempt.”
- “Our next goal was to accurately measure the asteroid’s position, but we had very few stars in our new image to use as a reference. There were 17 stars listed in an older catalogue and only four stars measured by Gaia. I made calculations using both sets of data.”
- “Later in the year, when the asteroid had been observed multiple times by other teams and its trajectory was better known, it became clear that the measurements I made using just four Gaia stars had been much more accurate than the ones using the 17 stars. This was really amazing.”
Keeping Earth Safe
- This same technique is being applied to asteroids that were never lost, allowing researchers to use data from Gaia to determine their trajectories and physical properties more accurately than ever before.
- This is helping them update asteroid population models and deepen our understanding of how asteroid orbits develop, for example, by measuring subtle dynamical effects that play a key role in pushing small asteroids into orbits that could see them collide with Earth.
Dancing with Daylight
- In order to make such accurate measurements of the positions of other stars, Gaia has a complicated relationship with our own.
- Gaia orbits around the second Lagrange point, L2, of the Sun-Earth system. This location keeps the Sun, Earth and Moon all behind Gaia, allowing it to observe a large portion of the sky without their interference. It is also in an even thermal radiation environment and experiences a stable temperature.
- However, Gaia must not fall entirely into Earth's shadow, as the spacecraft still depends on solar power. As the orbit around the L2 point is unstable, small disturbances can build up and see the spacecraft heading for an eclipse.

- Gaia's flight control team at ESA's ESOC mission control center in Darmstadt are responsible for making corrections to the spacecraft's trajectory to keep it in the correct orbit and out of Earth's shadow. They ensure that Gaia remains one of the most stable and accurate spacecraft ever. On 16 July 2019, the team successfully performed a crucial eclipse avoidance maneuver, moving Gaia into the extended phase of its mission and allowing it to keep scanning the sky for several more years.
• June 5, 2020: Chance of finding young Earth-like planets higher than previously thought, say Sheffield scientists. The team studied groups of young stars in the Milky Way to see if these groups were typical compared to theories and previous observations in other star-forming regions in space, and to study if the populations of stars in these groups affected the likelihood of finding forming Earth-like planets. 64)
a) New research from the University of Sheffield has found that the chance of finding earth-like planets in their early formation is much higher than previously thought
b) The team of researchers and undergraduate students studied these young Earth-like planets called magma ocean planets
c) The research will be vital to understanding how habitable planets like Earth form.

- The research, published in The Astrophysical Journal, found that there are more stars like the Sun than expected in these groups, which would increase the chances of finding Earth-like planets in their early stages of formation. 65)
- In their early stages of formation these Earth-like planets, called magma ocean planets, are still being made from collisions with rocks and smaller planets, which causes them to heat up so much that their surfaces become molten rock.
- The team, led by Dr Richard Parker, included undergraduate students from the University of Sheffield giving them the opportunity to apply the skills learnt on their course to leading published research in their field.
- Dr Richard Parker, from the University of Sheffield's Department of Physics and Astronomy, said: "These magma ocean planets are easier to detect near stars like the Sun, which are twice as heavy as the average mass star. These planets emit so much heat that we will be able to observe the glow from them using the next generation of infrared telescopes.
- "The locations where we would find these planets are so-called ‘young moving groups' which are groups of young stars that are less than 100 million years old - which is young for a star. However, they typically only contain a few tens of stars each and previously it was difficult to determine whether we had found all of the stars in each group because they blend into the background of the Milky Way galaxy.
- "Observations from the Gaia telescope have helped us to find many more stars in these groups, which enabled us to carry out this study."
- The findings from the research will help further understanding of whether star formation is universal and will be an important resource for studying how rocky, habitable planets like Earth form. The team now hopes to use computer simulations to explain the origin of these young moving groups of stars.
- The research team included undergraduate students Amy Bottrill, Molly Haigh, Madeleine Hole and Sarah Theakston from the University of Sheffield's Department of Physics and Astronomy.
- The team said: "Being involved in this project was one of the highlights of our university experience and it was a great opportunity to work on an area of astronomy outside the typical course structure.
- "It was rewarding to see a physical application of the computer coding we learnt in our degree by sampling the initial mass distribution of stars and how this can relate to the future of exoplanet detection."
- The Department of Physics and Astronomy at the University of Sheffield explores the fundamental laws of the universe and develops pioneering technologies with real-world applications. Researchers are looking beyond our planet to map out distant galaxies, tackling global challenges including energy security, and exploring the opportunities presented by quantum computing and 2D materials.
• June 4, 2020: An artificial intelligence system analyzing data from the Gaia space telescope has identified more than 2,000 large protostars - and they could hold clues to the origins of the stars in the Milky Way. 66)
- Protostars are young stars that are still forming. Scientists had previously catalogued only 100 of this type of forming star.
- The project was led by Miguel Vioque, a PhD researcher at the University of Leeds, and the findings have been published in the journal Astronomy and Astrophysics. 67)
- He believes investigation of these newly identified stars has the potential to change scientists' understanding of massive star formation and their approach to studying the galaxy.
- Mr Vioque and his colleagues were interested in what are known as Herbig Ae/Be stars, stars that have a mass that is at least twice that of the Sun. They are also involved in the birth of other stars.
- The researchers took the vast quantity of data being collected by the Gaia spaceborne telescope as it maps the galaxy. Launched in 2013, data collected by the telescope has enabled distances to be determined for about one billion stars, about one per cent of the total that are thought to exist in the galaxy.
- The researchers cleaned that data and reduced it to a subset of 4.1 million stars which were likely to contain the target protostars.
- The artificial intelligence (AI) system sifted the data and generated a list of 2,226 stars with around an 85 percent chance of being a Herbig Ae/Be protostar.
- Mr Vioque, from the School of Physics and Astronomy, said: "There is a huge amount of data being produced by Gaia – and AI tools are needed to help scientists make sense of it.
- "We are combining new technologies in the way researchers survey and map the galaxy with ways of interrogating the mountain of data produced by the telescope - and it is revolutionizing our understanding of the galaxy.
- "This approach is opening an exciting, new chapter in astronomy."
- Mr Vioque and his colleagues then validated the findings of the AI tool by investigating 145 of the stars identified by the AI system at ground observatories in Spain and Chile where they were able to measure the light, recorded as spectra, coming from the stars.
- He said: "The results from the ground-based observatories show that the AI tool made very accurate predictions about stars that were likely to fall into the Herbig Ae/Be classification."
- One of the target stars is known as Gaia DR2 428909457258627200.
- It is 8,500 light years away and has a mass 2.3 times that of the sun. Its surface temperature is 9,400 degrees Celsius – the sun is about 5,500 degrees Celsius – and it has a radius that is twice that of the sun. It has existed for around six million years, which in astronomical terms makes it a young star that is still forming.
- Professor René Oudmaijer, from the School of Physics and Astronomy at Leeds, supervised the research. He said: "This research is an excellent example of how the analysis of the Big Data collected by modern scientific instruments, such as the Gaia telescope, will shape the future of astrophysics.
- "AI systems are able to identify patterns in vast quantities of data – and it is likely that in those patterns, scientists will find clues that will lead to new discoveries and fresh understanding."
- The research was funded by the European Union's Horizon 2020 research and innovation program, under the STARRY project.

• May 25, 2020: The formation of the Sun, the Solar System and the subsequent emergence of life on Earth may be a consequence of a collision between our galaxy, the Milky Way, and a smaller galaxy called Sagittarius, discovered in the 1990s to be orbiting our galactic home. 68)
- Astronomers have known that Sagittarius repeatedly smashes through the Milky Way's disc, as its orbit around the galaxy's core tightens as a result of gravitational forces. Previous studies suggested that Sagittarius, a so called dwarf galaxy, had had a profound effect on how stars move in the Milky Way. Some even claim that the 10,000 times more massive Milky Way's trademark spiral structure might be a result of the at least three known crashes with Sagittarius over the past six billion years. 69)
- A new study, based on data gathered by ESA's galaxy mapping powerhouse Gaia, revealed for the first time that the influence of Sagittarius on the Milky Way may be even more substantial. The ripples caused by the collisions seem to have triggered major star formation episodes, one of which roughly coincided with the time of the formation of the Sun some 4.7 billion years ago.
- "It is known from existing models that Sagittarius fell into the Milky Way three times – first about five or six billion years ago, then about two billion years ago, and finally one billion years ago," says Tomás Ruiz-Lara, a researcher in Astrophysics at the Instituto de Astrofísica de Canarias (IAC) in Tenerife, Spain, and lead author of the new study published in Nature Astronomy.
- "When we looked into the Gaia data about the Milky Way, we found three periods of increased star formation that peaked 5.7 billion years ago, 1.9 billion years ago and 1 billion years ago, corresponding with the time when Sagittarius is believed to have passed through the disc of the Milky Way."

Ripples on the Water
- The researchers looked at luminosities, distances and colors of stars within a sphere of about 6500 light years around the Sun and compared the data with existing stellar evolution models. According to Tomás, the notion that the dwarf galaxy may have had such an effect makes a lot of sense.
- "At the beginning you have a galaxy, the Milky Way, which is relatively quiet," Tomás says. "After an initial violent epoch of star formation, partly triggered by an earlier merger as we described in a previous study, the Milky Way had reached a balanced state in which stars were forming steadily. Suddenly, you have Sagittarius fall in and disrupt the equilibrium, causing all the previously still gas and dust inside the larger galaxy to slosh around like ripples on the water."
- In some areas of the Milky Way, these ripples would lead to higher concentrations of dust and gas, while emptying others. The high density of material in those areas would then trigger the formation of new stars.
- "It seems that not only did Sagittarius shape the structure and influenced the dynamics of how stars are moving in the Milky Way, it has also led to a build-up of the Milky Way," says Carme Gallart, a co-author of the paper, also of the IAC. "It seems that an important part of the Milky Way's stellar mass was formed due to the interactions with Sagittarius and wouldn't exist otherwise."

The Birth of the Sun
- The effects of Sagittarius on the structure and movement of stars in the Milky Way have been described previously, but the new findings for the first time show that the dwarf galaxy was likely directly responsible for the build-up of the stellar mass in the Milky Way. In fact, our parent star, the Sun, formed during the period in the wake of the first known collision. The scientists admit that it cannot be proven whether the particular cloud of dust and gas that gave rise to our parent star collapsed as a result of the collision with Sagittarius. It, however, seems possible that without the dwarf galaxy crossing paths with the Milky Way, Earth and life on it may not have been born.
- In fact, it seems possible that even the Sun and its planets would not have existed if the Sagittarius dwarf had not gotten trapped by the gravitational pull of the Milky Way and eventually smashed through its disc.
- "The Sun formed at the time when stars were forming in the Milky Way because of the first passage of Sagittarius," says Carme. "We don't know if the particular cloud of gas and dust that turned into the Sun collapsed because of the effects of Sagittarius or not. But it is a possible scenario because the age of the Sun is consistent with a star formed as a result of the Sagittarius effect."
- Every collision stripped Sagittarius of some of its gas and dust, leaving the galaxy smaller after each passage. Existing data suggest that Sagittarius might have passed through the Milky Way's disc again quite recently, in the last few hundred million years, and is currently very close to it. In fact, the new study found of a recent burst of star formation, suggesting a possible new and ongoing wave of stellar birth.
- According to ESA Gaia project scientist Timo Prusti, such detailed insights into the Milky Way's star formation history wouldn't be possible before Gaia, the star-mapping telescope launched in late 2013, whose two data releases in 2016 and 2018 revolutionized the study of the Milky Way.
- "Some determinations of star formation history in the Milky Way existed before based on data from ESA's early 1990s Hipparcos mission," says Timo. "But these observations were focused on the immediate neighborhood of the Sun. It wasn't really representative and so it couldn't uncover those bursts in star formation that we see now.
- "This is really the first time that we see a detailed star formation history of the Milky Way. It's a testament to the scientific power of Gaia that we have seen manifest again and again in countless ground-breaking studies in a period of only a couple of years." 70)
• April 25, 2020: Today the Gaia Mission celebrates the second anniversary of Gaia Data Release 2 (DR2). The Gaia catalogues have been embraced by many scientists across the world. Today, we are proud of the many papers that appeared using our latest release, Gaia DR2. In the past 2 years, almost 3000 refereed papers based on Gaia Data Release 2 have been published. That amounts on average to 4 papers per day. Thank you for using our data with so much enthusiasm! In the meantime, ESA Gaia teams and Gaia DPAC are continuously working towards next data releases. Keep posted with our Gaia newsletter of upcoming releases. 71)
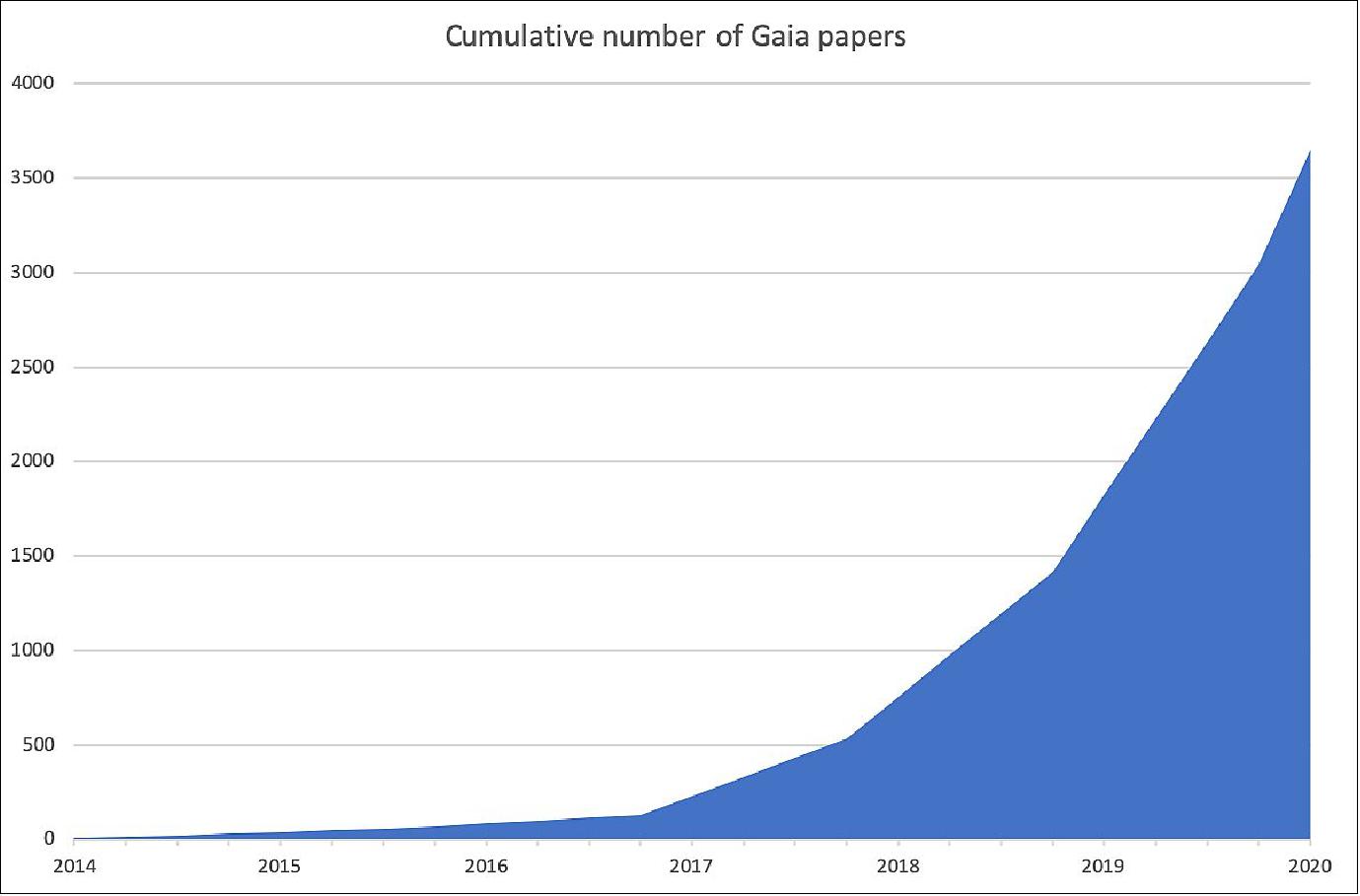
• March 20, 2020: The Gaia collaboration is currently involved in the production of Early Data Release 3 (EDR3), Data Release 3 and Data Release 4. While the data for Gaia Early Data Release 3 has been processed, it is currently being validated, documented and structured to appear in the Gaia Archive. In parallel the data is being processed further to create all data products to be released in Gaia Data Release 3. 72)
- At the same time, the Gaia spacecraft has just finished gathering the data that will be used for the release covering the full nominal mission plus the first 6 months of the mission extension (so covering a period of 66 months). This data will soon start running through the first pipelines in our software chain.
- These preparations for the data releases rely on a tight collaboration of many scientists and computer engineers across Europe and with the current measures in place to contain and mitigate the effects of the COVID-19 virus, a delay of Gaia (E)DR3 is therefore announced, as you can read in the below news item.
Delay of Gaia (E)DR3
- The COVID-19 virus is spreading across the globe and its impact is also felt in the Gaia collaboration. The data processing effort is distributed over many European countries which adopt different approaches to fight the pandemic. In some countries, restrictions are very severe and the situation is rapidly changing towards more restrictions everywhere.
- The schedule towards Gaia (E)DR3 is already affected and more delays can be anticipated. Those scientists and computer engineers in the Gaia DPAC (Data Processing and Analysis Consortium) who can work are now mostly working from home, but their priority is their own health and that of their families. Furthermore, resolving problems with computer hardware takes longer than usual due to the absence of personnel on operational sites.
- Therefore, schedule delays of both Gaia EDR3 and Gaia DR3 are inevitable, but can only be quantified after more clarity of the overall situation has been achieved. As soon as possible, a new schedule for the releases will be announced. While data processing has slowed down, the good news is that Gaia continues to collect valuable science data. With this note we wish everyone good health in the coming times.
Gaia DPAC Consortium Meeting Moved to 2021
- Also due to the COVID-19 virus, the Gaia DPAC consortium meeting that was planned to take place in March 2020 was replaced with an on-line meeting with a new physical meeting planned for March 2021.
• March 2, 2020: Astronomers have pondered for years why our galaxy, the Milky Way, is warped. Data from ESA's star-mapping satellite Gaia suggest the distortion might be caused by an ongoing collision with another, smaller, galaxy, which sends ripples through the galactic disc like a rock thrown into water. 73)

- Astronomers have known since the late 1950s that the Milky Way's disc – where most of its hundreds of billions of stars reside – is not flat but somewhat curved upwards on one side and downwards on the other. For years, they debated what is causing this warp. They proposed various theories including the influence of the intergalactic magnetic field or the effects of a dark matter halo, a large amount of unseen matter that is expected to surround galaxies. If such a halo had an irregular shape, its gravitational force could bend the galactic disc.
Faster than Expected
- With its unique survey of more than one billion stars in our galaxy, Gaia might hold the key to solving this mystery. A team of scientists using data from the second Gaia data release has now confirmed previous hints that this warp is not static but changes its orientation over time. Astronomers call this phenomenon precession and it could be compared to the wobble of a spinning top as its axis rotates.
- Moreover, the speed at which the warp precesses is much faster than expected – faster than the intergalactic magnetic field or the dark matter halo would allow. That suggests the warp must be caused by something else. Something more powerful – like a collision with another galaxy.
- "We measured the speed of the warp by comparing the data with our models. Based on the obtained velocity, the warp would complete one rotation around the center of the Milky Way in 600 to 700 million years," says Eloisa Poggio of the Turin Astrophysical Observatory, Italy, who is the lead author of the study, published in Nature Astronomy. "That's much faster than what we expected based on predictions from other models, such as those looking at the effects of the non-spherical halo." 74)
The Star Power of Gaia
- The warp's speed is, however, slower than the speed at which the stars themselves orbit the galactic center. The Sun, for example, completes one rotation in about 220 million years.
- Such insights were only possible thanks to the unprecedented ability of the Gaia mission to map our galaxy, the Milky Way, in 3D, by accurately determining positions of more than one billion stars in the sky and estimating their distance from us. The flying saucer-like telescope also measures the velocities at which individual stars move in the sky, allowing astronomers to ‘play' the movie of the Milky Way's history back- and forward in time over millions of years.
- "It's like having a car and trying to measure the velocity and direction of travel of this car over a very short period of time and then, based on those values, trying to model the past and future trajectory of the car," says Ronald Drimmel, a research astronomer at the Turin Astrophysical Observatory and co-author of the paper. "If we make such measurements for many cars, we could model the flow of traffic. Similarly, by measuring the apparent motions of millions of stars across the sky we can model large scale processes such as the motion of the warp."
Sagittarius?
- The astronomers do not yet know which galaxy might be causing the ripple nor when the collision started. One of the contenders is Sagittarius, a dwarf galaxy orbiting the Milky Way, which is believed to have burst through the Milky Way's galactic disc several times in the past. Astronomers think that Sagittarius will be gradually absorbed by the Milky Way, a process which is already underway.
- "With Gaia, for the first time, we have a large amount of data on a vast amount stars, the motion of which is measured so precisely that we can try to understand the large scale motions of the galaxy and model its formation history," says ESA's Gaia deputy project scientist Jos de Bruijne. "This is something unique. This really is the Gaia revolution."
- As impressive as the warp and its precession appear on the galactic scale, the scientists reassure us that it has no noticeable effects on life on our planet.

Far Enough
- "The Sun is at the distance of 26 000 light years from the galactic center where the amplitude of the warp is very small," Eloisa says. "Our measurements were mostly dedicated to the outer parts of the galactic disc, out to 52 000 light years from the galactic center and beyond."
- Gaia previously uncovered evidence of collisions between the Milky Way and other galaxies in the recent and distant past, which can still be observed in the motion patterns of large groups of stars billions of years after the events occurred.
- Meanwhile, the satellite, currently in the sixth year of its mission, keeps scanning the sky and a Europe-wide consortium is busy processing and analyzing the data that keeps flowing towards Earth. Astronomers across the world are looking forward to the next two Gaia data releases, planned for later in 2020 and in the second half 2021, respectively, to tackle further mysteries of the galaxy we call home.

• February 13, 2020: A sizable international team published findings about the discovery of a new binary star in Astronomy & Astrophysics (Ref. 79). A co-author from Kazan Federal University of the Russian Federation, Professor, Corresponding Member of the Tatarstan Academy of Sciences, Chair of the Department of Astronomy and Space Geodesy Ilfan Bikmaev, explains how the new system was found. 75)

- "The gravitational lensing method is one of the most powerful space exploration tools. In space, photons deviate from the rectilinear direction when passing near a massive body (star) under the influence of its gravitational field. If we take as a lens a celestial body, which is a sphere, then it will bend the space spherically symmetrically. However, the gravitational fields of many space objects do not have spherical symmetry, so more complex curvatures may appear. After their path has been curved, the photons will be summed up with those that hit the receiver earlier, and, as a result, an increase in the brightness of the star will occur. As a result, an increase in the brightness of the object is displayed on the light curve of the source, and this increase is not associated with a change in the physical parameters of the source itself.
- "If between a star of our Galaxy and an observer on Earth a massive object (a star-lens) moves across the line of sight, then when the lens passes exactly upon the line of sight, the effect of gravitational lensing will manifest itself in the form of a short-term (hours to days) brightening of the background star. Such events are called gravitational microlensing events. They are quite rare, isolated, short-lived and unpredictable."
- As the interviewee, in order to register a microlensing event in the Milky Way, you need to track the brilliance of hundreds of millions of stars daily. In particular, the space mission of the European Space Agency (GAIA) is engaged in this. Any brightness changes amounting to tens of percents from celestial sources that fall into the field of view of the GAIA space observatory are reported to Earth. And then the international network of telescopes around the globe begins to track these objects and identify the nature of variability.
- "Since 2016, astronomers of Kazan Federal University, together with Turkish colleagues, have been participating in the GAIA satellite object classification program. The vast majority of variable objects are cataclysmic variables, some are supernovae, and some are active galactic nuclei, which change their brightness from time to time. But there are objects that, while not being a variable, change their brightness for a short period of time, and then it attenuates. Such cases are unique," says Bikmaev. "So, in August 2016, the GAIA satellite discovered an object that received the designation Gaia16aye, the brightness change of which exceeded the accuracy of registration of the telescope and continued to increase. Turkish colleagues, analyzing the nature of the brightness change, suggested that this is not a variable object, but the microlensing effect. Polish colleagues, experts in the field of research on the effects of microlensing, organized an international campaign on photometry of this source, which was soon joined by Kazan Federal University. Observations of this unique object were carried out both in Turkey with the RTT 150 telescope and at the North Caucasian Astronomical Station.
- "The data obtained make it possible for the first time to simulate a situation where an observer on Earth makes a yearly motion around the Sun, a gravitating body moves in the form of a binary system around the center of mass, and the binary system has its own motion in the Galaxy. This is a rather complex kinematic movement. Therefore, the system of these maxima is complex. And what we can do is accurately measure the brightness change.
- "With a single passage, a single maximum is observed, and then the brightness curve of the object drops to the initial level. In the case of the Gaia16aye event, after the first maximum, the light curve did not drop to the initial level. Therefore, astronomers have made the assumption that the gravitational lens is not a single object, but a binary system. And then the third peak appeared and everyone understood that it was, without a doubt, a binary system. Perhaps the geometry of the system is even more complex. In this article, a group of Polish scientists, based on international cooperative observations and their own theoretical calculations, built a geometric picture of the occurrence of the Gaia16aye microlensing phenomenon," concludes Professor Bikmaev.
• February 10, 2020: Scientists from Rochester Institute of Technology have discovered a newborn massive planet closer to Earth than any other of similarly young age found to date. The baby giant planet, called 2MASS 1155-7919 b, is located in the Epsilon Chamaeleontis Association and lies only about 330 light years from our solar system. 76)

- The discovery, published in the Research Notes of the American Astronomical Society, provides researchers an exciting new way to study how gas giants form.
- "The dim, cool object we found is very young and only 10 times the mass of Jupiter, which means we are likely looking at an infant planet, perhaps still in the midst of formation," said Annie Dickson-Vandervelde, lead author and astrophysical sciences and technology Ph.D. student from West Columbia, S.C. "Though lots of other planets have been discovered through the Kepler mission and other missions like it, almost all of those are ‘old' planets. This is also only the fourth or fifth example of a giant planet so far from its ‘parent' star, and theorists are struggling to explain how they formed or ended up there."
- The scientists used data from the Gaia space observatory to make the discovery. The giant baby planet orbits a star that is only about 5 million years old, about one thousand times younger than our sun. The planet orbits its sun at 600 times the distance of the Earth to the sun. How this young, giant planet could have ended up so far away from its young "parent" star is a mystery. The authors hope that follow-up imaging and spectroscopy will help astronomers understand how massive planets can end up in such wide orbits.
• January 21, 2020: A 500-day global observation campaign spearheaded more than three years ago by ESA's galaxy-mapping powerhouse Gaia has provided unprecedented insights into the binary system of stars that caused an unusual brightening of an even more distant star. 77)

- The brightening of the star, located in the Cygnus constellation, was first spotted in August 2016 by the Gaia Photometric Science Alerts program.
- This system, maintained by the Institute of Astronomy at the University of Cambridge, UK, scans daily the huge amount of data coming from Gaia and alerts astronomers to the appearance of new sources or unusual brightness variations in known ones, so that they can quickly point other ground and space-based telescopes to study them in detail. The phenomena may include supernova explosions and other stellar outbursts.
- In this particular instance, follow-up observations performed with more than 50 telescopes worldwide revealed that the source – since then named Gaia16aye after Ayers Rock, the famous landmark in Australia – was behaving in a rather strange way.
- "We saw the star getting brighter and brighter and then, within one day, its brightness suddenly dropped," says Lukasz Wyrzykowski from the Astronomical Observatory at the University of Warsaw, Poland, who is one of the scientists behind the Gaia Photometric Science Alert program.
- "This was a very unusual behavior. Hardly any type of supernova or other star does this."
- Lukasz and collaborators soon realized that this brightening was caused by gravitational microlensing – an effect predicted by Einstein's theory of general relativity, caused by the bending of spacetime in the vicinity of very massive objects, like stars or black holes.
- When such a massive object, which may be too faint to be observed from Earth, passes in front of another, more distant source of light, its gravity bends the fabric of spacetime in its vicinity. This distorts the path of light rays coming from the background source – essentially behaving like a giant magnifying glass. — Gaia16aye is the second micro-lensing event detected by ESA's star surveyor. However, the astronomers noticed it behaved strangely even for this type of event.
- "If you have a single lens, caused by a single object, there would be just a small, steady rise in brightness and then there would be a smooth decline as the lens passes in front of the distant source and then moves away," says Lukasz.
- "In this case, not only did the star brightness drop sharply rather than smoothly, but after a couple of weeks it brightened up again, which is very unusual. Over the 500 days of observation, we have seen it brighten up and decline five times."

- This sudden and sharp drop in brightness suggested that the gravitational lens causing the brightening must consist of a binary system – a pair of stars, or other celestial objects, bound to one another by mutual gravity.
- The combined gravitational fields of the two objects produce a lens with a rather intricate network of high magnification regions. When a background source passes through such regions on the plane of the sky, it lights up, and then dims immediately upon exiting it.
- From the pattern of subsequent brightenings and dimmings, the astronomers were able to deduce that the binary system was rotating at a rather fast pace.
- "The rotation was fast enough and the overall micro-lensing event slow enough that the background star entered the high magnification region, left it and then entered it again," says Lukasz.
- The long period of observations, which lasted until the end of 2017, and the extensive participation of ground-based telescopes from around the globe enabled the astronomers to gather a large amount of data – almost 25,000 individual data points.
- In addition, the team also made use of dozens of observations of this star collected by Gaia as it kept scanning the sky over the months. These data have undergone preliminary calibration and were made public as part of the Gaia Science Alerts program.
- From this data set, Lukasz and his colleagues were able to learn a great deal of detail about the binary system of stars.
- "We don't see this binary system at all, but from only seeing the effects that it created by acting as a lens on a background star, we were able to tell everything about it," says co-author Przemek Mróz, who was a PhD student at the University of Warsaw when the campaign started, and is currently a postdoctoral scholar at the California Institute of Technology.
- "We could determine the rotational period of the system, the masses of its components, their separation, the shape of their orbits – basically everything – without seeing the light of the binary components."
- The pair consists of two rather small stars, with 0.57 and 0.36 times the mass of our Sun, respectively. Separated by roughly twice the Earth-Sun distance, the stars orbit around their mutual center of mass in less than three years.
- "If it wasn't for Gaia scanning the whole sky and then sending the alerts straight away, we would never have known about this microlensing event," says co-author Simon Hodgkin from the University of Cambridge, who leads the Gaia Science Alerts program. - "Maybe we would have found it later, but then it might have been too late."
- The detailed understanding of the binary system relied on the extensive observation campaign and on the broad international involvement that the Gaia16aye event attracted.
- "We acknowledge the professional astronomers, amateur astronomers and volunteers from all around the globe who have been observing this event: without the dedication of all those people we wouldn't have been able to obtain such results," says Lukasz.
- "Microlensing events like this can shed light on celestial objects that we would otherwise not be able to see," says Timo Prusti, Gaia Project Scientist at ESA. "We are delighted that Gaia's detection triggered the observation campaign that made this result possible." 79)
• January 7, 2020: Astronomers at Harvard University have discovered a monolithic, wave-shaped gaseous structure — the largest ever seen in our galaxy — made up of interconnected stellar nurseries. Dubbed the "Radcliffe Wave" in honor of the collaboration's home base, the Radcliffe Institute for Advanced Study, the discovery transforms a 150-year-old vision of nearby stellar nurseries as an expanding ring into one featuring an undulating, star-forming filament that reaches trillions of miles above and below the galactic disk. 80)
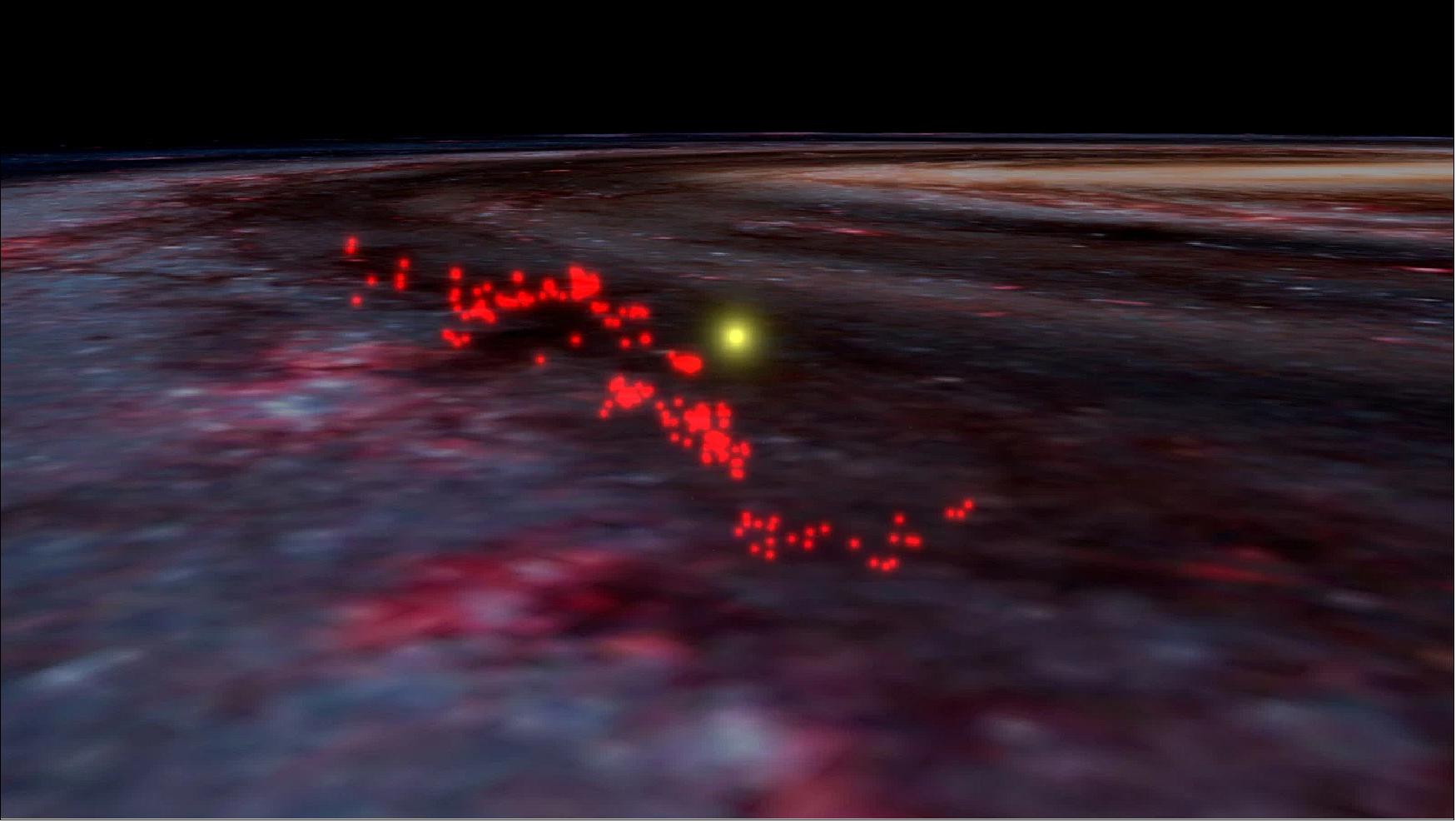
- The work, published in Nature, was enabled by a new analysis of data from the European Space Agency's Gaia spacecraft, launched in 2013 with the mission of precisely measuring the position, distance, and motion of the stars. The research team's innovative approach combined the super-accurate data from Gaia with other measurements to construct a detailed, 3D map of interstellar matter in the Milky Way, and noticed an unexpected pattern in the spiral arm closest to Earth. 81)
- The researchers discovered a long, thin structure, about 9,000 light-years long and 400 light-years wide, with a wave-like shape, cresting 500 light-years above and below the mid-plane of our galaxy's disk. The Wave includes many of the stellar nurseries that were thought to form part of "Gould's Belt," a band of star-forming regions believed to be oriented in a ring around the sun.
- "No astronomer expected that we live next to a giant, wave-like collection of gas — or that it forms the local arm of the Milky Way," said Alyssa Goodman, the Robert Wheeler Willson Professor of Applied Astronomy, research associate at the Smithsonian Institution, and co-director of the Science Program at the Radcliffe Institute for Advanced Study. "We were completely shocked when we first realized how long and straight the Radcliffe Wave is, looking down on it from above in 3D — but how sinusoidal it is when viewed from Earth. The Wave's very existence is forcing us to rethink our understanding of the Milky Way's 3D structure."
- "Gould and Herschel both observed bright stars forming in an arc projected on the sky, so for a long time, people have been trying to figure out if these molecular clouds actually form a ring in 3D," said João Alves, a professor of physics and astronomy at the University of Vienna and 2018‒2019 Radcliffe Fellow. "Instead, what we've observed is the largest coherent gas structure we know of in the galaxy, organized not in a ring but in a massive, undulating filament. The sun lies only 500 light-years from the Wave at its closest point. It's been right in front of our eyes all the time, but we couldn't see it until now."

- The new, 3D map shows our galactic neighborhood in a new light, giving researchers a revised view of the Milky Way and opening the door to other major discoveries.
- "We don't know what causes this shape, but it could be like a ripple in a pond, as if something extraordinarily massive landed in our galaxy," said Alves. "What we do know is that our sun interacts with this structure. It passed by a festival of supernovae as it crossed Orion 13 million years ago, and in another 13 million years it will cross the structure again, sort of like we are ‘surfing the wave.'"
- Disentangling structures in the "dusty" galactic neighborhood within which we sit is a longstanding challenge in astronomy. In earlier studies, the research group of Douglas Finkbeiner, professor of astronomy and physics at Harvard, pioneered advanced statistical techniques to map the 3D distribution of dust using vast surveys of stars' colors. Armed with new data from Gaia, Harvard graduate students Catherine Zucker and Joshua Speagle recently augmented these techniques, dramatically improving astronomers' ability to measure distances to star-forming regions. That work, led by Zucker, is published in the Astrophysical Journal.
- "We suspected there might be larger structures that we just couldn't put in context. So, to create an accurate map of our solar neighborhood, we combined observations from space telescopes like Gaia with astrostatistics, data visualization, and numerical simulations," explained Zucker, a National Science Foundation graduate fellow and a Ph.D. candidate in the Department of Astronomy at Harvard's Graduate School of Arts and Sciences.
- "The sun lies only 500 light-years from the Wave at its closest point. It's been right in front of our eyes all the time, but we couldn't see it until now," according to João Alves, Radcliffe Fellow 2018-19.
- Zucker played a key role in compiling the largest-ever catalog of accurate distances to local stellar nurseries — the basis for the 3D map used in the study. She has set herself the goal of painting a new picture of the Milky Way, near and far.
- "We pulled this team together so we could go beyond processing and tabulating the data to actively visualizing it — not just for ourselves but for everyone. Now, we can literally see the Milky Way with new eyes," she said.
- "Studying stellar births is complicated by imperfect data. We risk getting the details wrong, because if you're confused about distance, you're confused about size," said Finkbeiner.
- Goodman agreed, "All of the stars in the universe, including our sun, are formed in dynamic, collapsing, clouds of gas and dust. But determining how much mass the clouds have, how large they are, has been difficult, because these properties depend on how far away the cloud is."
- According to Goodman, scientists have been studying dense clouds of gas and dust between the stars for more than 100 years, zooming in on these regions with ever-higher resolution. Before Gaia, there was no data set expansive enough to reveal the galaxy's structure on large scales. Since its launch in 2013, the space observatory has enabled measurements of the distances to one billion stars in the Milky Way.
- The flood of data from Gaia served as the perfect testbed for innovative, new statistical methods that reveal the shape of local stellar nurseries and their connection to the Milky Way's galactic structure. Alves came to Radcliffe to work with Zucker and Goodman, as they anticipated the flood of data from Gaia would enhance the Finkbeiner group's "3D Dust Mapping" technology enough to reveal the distances of local stellar nurseries. But they had no idea they would find the Radcliffe Wave.
- The Finkbeiner, Alves, and Goodman groups collaborated closely on this data-science effort. The Finkbeiner group developed the statistical framework needed to infer the 3D distribution of the dust clouds; the Alves group contributed deep expertise on stars, star formation, and Gaia; and the Goodman group developed the 3D visualizations and analytic framework, called "glue," that allowed the Radcliffe Wave to be seen, explored, and quantitatively described.
- This study was supported by the NSF Graduate Research Fellowship Program (grant no. 1650114, AST-1614941), the Harvard Data Science Initiative, NASA through ADAP (grant no. NNH17AE75I), and a Hubble Fellowship (grant HST-HF2-51367.001-A) awarded by the Space Telescope Science Institute, which is operated by the Association of Universities for Research in Astronomy, Inc., for NASA, under contract NAS 5-26555.
PLM (Payload Module)
The payload module is housed inside a geometrical, dome-like structure called the 'Thermal Tent' (Figures 14 and 15). The payload consists of a single integrated instrument (Figure 82) that comprises three major functions. In the earlier spacecraft designs, the three functions were distributed over three separate instruments. Now the three functions are built into a single instrument by using common telescopes and a shared focal plane: 82) 83) 84) 85) 86)
1) The Astrometric instrument (ASTRO) is devoted to star angular position measurements, providing the five astrometric parameters:
- Star position (2 angles)
- Proper motion (2 time derivatives of position)
- Parallax (distance)
ASTRO is functionally equivalent to the main Hipparcos instrument.
2) The Photometric instrument provides continuous star spectra for astrophysis in the band 320-1000 nm and the ASTRO chromaticity calibration
3) The RVS (Radial Velocity Spectrometer) provides radial velocity and high resolution spectral data in the narrow band 847-874 nm.
Each function is achieved within a dedicated area on the focal plane. Afocal elements are located close to the focal plane for the photometric and spectroscopic functions, providing dispersion of the star's spectrum along the scan. This allows both functions to take benefit from the two viewing directions and from the large ASTRO aperture, and to operate in densely populated sky areas. RVS is implemented as a grating plate, combined with four prismatic spherical lenses. This allows the necessary dispersion value to be met while correcting most of the telescope aberrations. 87)

Legend to Figure 82: The focal plane is hanging on the ‘optical bench torus' made of silicon carbide. The optics consist of 10 mirrors and the refractive optical elements. Mirrors M1, M2 and M3 form one telescope and M1', M2', M3' the other telescope. The subsequent set of mirrors M4/M4', M5 and M6 combine the light from both telescopes and direct it to the focal plane assembly. The fields of view of the two telescopes are 106.5º apart (Astrium SAS).
Design:
The payload design is characterized by:
• A dual telescope concept, with a common structure and a common focal plane. Both telescopes are based on a TMA (Three Mirror Anastigmat) design. The beam combination is achieved in image space with a small beam combiner, rather than in object space as was done in the Hipparcos satellite. This saves the mass of the beam combiner, simplifies the accommodation and eliminates the directional ambiguity of the detected targets.
• The use of SiC (Silicon Carbide) ultra-stable material for mirrors and telescope structure provides low mass, isotropy, thermo-elastic stability and dimensional stability in a space environment. This allows to meet the stability requirements for the basic angle between the two telescopes with a passive thermal control instead of an active one.
• A highly robust BAM (Basic Angle Measurement) system.
• A large common focal plane shared by all instruments.
Optics:
Gaia contains two identical telescopes, pointing in two directions separated by a 106.5º basic angle and merged into a common path at the exit pupil. The optical path of both telescopes is composed of six reflectors (M1-M6), the last two of which are common (M5-M6). Both telescopes have an aperture of 1.45 m x 0.5 m and a focal length of 35 m. The telescope elements are built around the hexagonal optical bench with a ~3 m diameter, which provides the structural support.

Although the optical design is fully reflective, based on mirrors only, diffraction effects with residual aberrations induce systematic chromatic shifts of the diffraction images and thus of the measured star positions. This effect, usually neglected in optical systems, is also critical for Gaia. These systematic chromatic displacements will be calibrated as part of the on-ground data analysis using the color information provided by the photometry of each observed object.
Astrometry
The main objective of the astrometric instrument (ASTRO) is to obtain accurate measurements of the relative positions of all objects that cross the fields of view of Gaia's two telescopes. The two fields of view are combined onto the single focal plane.
During its five-year mission, Gaia will systematically scan the whole sky and will have obtained some 70 sets of relative position measurements for each star. These permit a complete determination of each star's five basic astrometric parameters: two specifying the angular position, two specifying the proper motion, and one - the parallax - specifying the star's distance. The five-year long mission also permits the determination of additional parameters, for example those relevant to orbital binaries, extra-solar planets, and solar-system objects.
By measuring the instantaneous image centroids from the data sent to ground, Gaia measures the relative separations of the thousands of stars simultaneously present in the combined two fields. The spacecraft operates in a continuously scanning motion, such that a constant stream of relative angular measurements is built up as the fields of view sweep across the sky. High angular resolution (and hence high positional precision) in the scanning direction is provided by the large primary mirror of each telescope. The wide-angle measurements provide high rigidity of the resulting reference system.
Design: The astrometric instrument (ASTRO) comprises the two telescopes and the dedicated area of 62 CCDs in the focal plane, where the two fields of view are combined onto the AF (Astrometic Field). Each CCD is read out in TDI (Time Delay Integration) mode, synchronized to the scanning motion of the satellite. In practice, stars entering the combined field of view first pass across the column of the SM (Sky Mapper) CCDs, where each object is detected. Information on an object's position and brightness is processed on board in real-time to define the windowed region around the object to be read out by the following CCDs.
On-ground Data Processing: The a posteriori on-ground data processing is a highly complex task, linking all relative measurements and transforming the location (centroiding) measurements in pixel coordinates to angular field coordinates through a geometrical calibration of the focal plane, and subsequently to coordinates on the sky through calibrations of the instrument attitude and basic angle.
Further necessary corrections to be performed include those for optics effects (systematic chromatic shifts and aberration) and general-relativistic effects (light bending due to the Sun, the major planets plus some of their moons, and the most massive asteroids).
Accuracy: The accuracy of the measurements depends on the stellar type and relies on the stability of the basic angle of 106.5° between the two telescopes. This angle is monitored by the BAM (Basic Angle Monitoring) system.
Photometry
The photometer will measure the SED (Spectral Energy Distribution) of all the detected objects. This will serve two goals:
• From the SED measurements, astrophysical quantities such as luminosity, effective temperature, mass, age, and chemical composition are derived
• In order to meet the astrometric performance requirements, the measured centroid positions must be corrected for systematic chromatic shifts induced by the optical system. This is only possible with the knowledge of the spectral energy distribution of each observed target in the wavelength range covered by the CCDs of the main astrometric field (~320-1000 nm).
Design: The photometer (like the spectrometer) is merged with the astrometric function, using the same large collecting apertures of the two telescopes. The photometry function is achieved by means of two low dispersion optics located in the common path of the two telescopes: one for the short wavelengths (BP) and one for the long wavelengths (RP).
- BP (Blue Photometer): Measurement in the 320-660 nm spectral range
- RP (Red Photometer): Measurement in the 650-1000 nm spectral range.
The baseline design uses only one prismatic element in fused silica for each photometer, to disperse the collected light along scan prior to detection.
The prisms are located at the nearest possible position from the focal plane, in order to facilitate the mechanical holding and moreover reduce the shadowed areas. Both prisms are attached to the box shaped CCD radiator directly in front of the detector array. Both photometers, BP and RP, have a dedicated CCD strip that covers the full astrometric field of view in the across-scan direction.
Accuracy: The spectral resolution is a function of wavelength as a result of the natural dispersion curve of fused silica; the dispersion is higher at short wavelengths.
The BP and RP dispersers will be designed in such a way that BP and RP spectra have similar sizes (on the order of 30 pixels along scan). BP and RP spectra will be binned on-chip in the across-scan direction; no along-scan binning is foreseen. For bright stars, single-pixel-resolution windows are foreseen to be used, in combination with TDI gates.
The end of mission sky averaged magnitude standard error will depend on the star type, magnitude and wavelength band and it will typically be in the range of 10-200 x 10-3 in magnitude.
Spectrometry
Objectives: The primary objective of Gaia's RVS (Radial Velocity Spectrometer) instrument is the acquisition of radial velocities. These LOS (Line-of-Sight) velocities complement the proper-motion measurements provided by the astrometric instrument. To this end, the instrument will obtain spectra in the narrow near infrared band (847-874 nm) with a spectral resolution λ/Δλ of ~ 11,500.
The RVS wavelength range, 847-874 nm, has been selected to coincide with the energy-distribution peaks of G- and K-type stars which are the most abundant RVS targets. For these late-type stars, the RVS wavelength interval displays, besides numerous weak lines mainly due to Fe, Si, and Mg, three strong ionized calcium lines (at around 849.8, 854.2, and 855.2 nm). The lines in this triplet allow radial velocities to be derived, even at modest SNRs (Signal-to-Noise Ratios). In early-type stars, the RVS spectra may contain weak lines such as Ca II, He I, He II, and N I, although they will generally be dominated by Hydrogen Paschen lines.
Design and operations: The RVS instrument is a near-infrared, medium-resolution, integral-field spectrograph dispersing all the light entering the field of view. It is integrated with the astrometric and photometric functions and uses the common two telescopes.
- Wavelength range: 847 - 874 nm
- Resolution (R=λ/Δλ): ~ 11,500.
The RVS uses the SM (Sky Mapper) function for object detection and confirmation. Objects will be selected for RVS observations, based on measurements made slightly earlier in the RP (Red Photometer). Light from objects coming from the two viewing directions of the two telescopes is superimposed on the RVS CCDs.

The spectral dispersion of objects in the field of view is achieved by means of an optical module physically located between the last telescope mirror (M6) and the focal plane. This module contains a grating plate and four dioptric, prismatic, spherical, fused-silica lenses which correct the main aberrations of the off-axis field of the telescope. The RVS module has unit magnification, which means that the effective focal length of the RVS equals 35 m.
Spectral dispersion is oriented in the along-scan direction. A dedicated passband filter restricts the throughput of the RVS to the desired wavelength range.
The RVS-part of the Gaia FPA (Focal Plane Assembly) contains 3 CCD strips and 4 CCD rows. Each source will typically be observed during ~40 FOV transits throughout the 5-year mission. On the sky, the RVS CCD rows are aligned with the astrometric and photometric CCD rows; the resulting semi-simultaneity of the astrometric, photometric, and spectroscopic transit data will be advantageous for variability analyses, scientific alerts, spectroscopic binaries, etc. All RVS CCDs are operated in TDI (Time Delay Integration) mode.
The RVS spectra will be binned on-chip in the across-scan direction. All single-CCD spectra are foreseen to be transmitted to the ground without any further on-board (pre-)processing. For bright stars, single-pixel-resolution windows are foreseen to be used, possibly in combination with TDI gates. It is currently foreseen that the RVS will be able to reach object densities on the sky of up to 40,000 objects/ degree2.
On-ground Data Processing: Radial velocities will be obtained by cross-correlating observed spectra with either a template or a mask. An initial estimate of the source atmospheric parameters derived from the astrometric and photometric data will be used to select the most appropriate template or mask. Iterative improvements of this procedure are foreseen. For stars brighter than ~15th magnitude, it will be possible to derive radial velocities from spectra obtained during a single field-of-view transit. For fainter stars, down to ~17th magnitude, accurate summation of the ~40 transit spectra collected during the mission will allow the determination of mean radial velocities.
Atmospheric parameters will be extracted from observed spectra by comparison of the latter to a library of reference-star spectra using, for example, minimum-distance methods, principal-component analyses, or neural-network approaches. The determination of the source parameters will also rely on the information collected by the other two instruments: astrometric data will constrain surface gravities, while photometric observations will provide information on many astrophysical parameters.


As the spacecraft slowly rotates, the light from the celestial object (that is, the image of the object) passes across the focal plane. In this way, Gaia steadily scans the whole sky as the satellite spins and gradually precesses, with each part being observed around 70 times in the course of the operational lifetime.
FPA (Focal Plane Assembly) 88) 89)
The Gaia focal-plane assembly is the largest ever developed for a space application, with 106 CCDs, a total of 937 Mpixels (almost 1 Gpixel) , which are around 90% light efficient (c.f. 20% typical terrestrial camera efficiency). The FPA has a physical dimension of 1.0 m x 0.4 m (Figure 87). The focal-plane assembly is common to both telescopes and serves five main functions:
1) The WFS (Wave-Front Sensor) and basic-angle monitor, covering 2+2 CCDs: a five-degrees-of-freedom mechanism is implemented behind the M2/M2' secondary mirrors of the two telescopes for re-aligning the telescopes in orbit to cancel errors due to mirror micro-settings and gravity release. These devices are activated following the output of two Shack–Hartmann-type wave-front sensors at different positions in the focal plane. The BAM (Basic Angle Monitor) system (2 CCDs in cold redundancy) consists of a Youngs-type interferometer continuously measuring fluctuations in the basic angle between the two telescopes with a resolution of 0.5 µarcsec per 15 minutes.
2) The SM (Sky Mapper), containing 14 CCDs (seven per telescope), which autonomously detects objects down to 20th magnitude entering the fields of view and communicates details of the star transits to the subsequent CCDs.
3) The main AF (Astrometric Field), covering 62 CCDs, devoted to angular-position measurements, providing the five astrometric parameters: star position (two angles), proper motion (two time derivatives of position), and parallax (distance) of all objects down to 20th magnitude. The first strip of seven detectors (AF1) also serves the purpose of object confirmation.
4) The blue and red photometers (BP and RP), providing low-resolution, spectro-photometric measurements for each object down to 20th magnitude over the wavelength ranges 330–680 nm and 640–1050 nm, respectively. The data serves general astrophysics and enables the on-ground calibration of telescope-induced chromatic image shifts in the astrometry. The photometers contain seven CCDs each.
5) The RVS (Radial Velocity Spectrometer), covering 12 CCDs in a 3 x 4 arrangement, collecting high-resolution spectra of all objects brighter than 17th magnitude, allowing derivation of radial velocities and stellar atmospheric parameters.
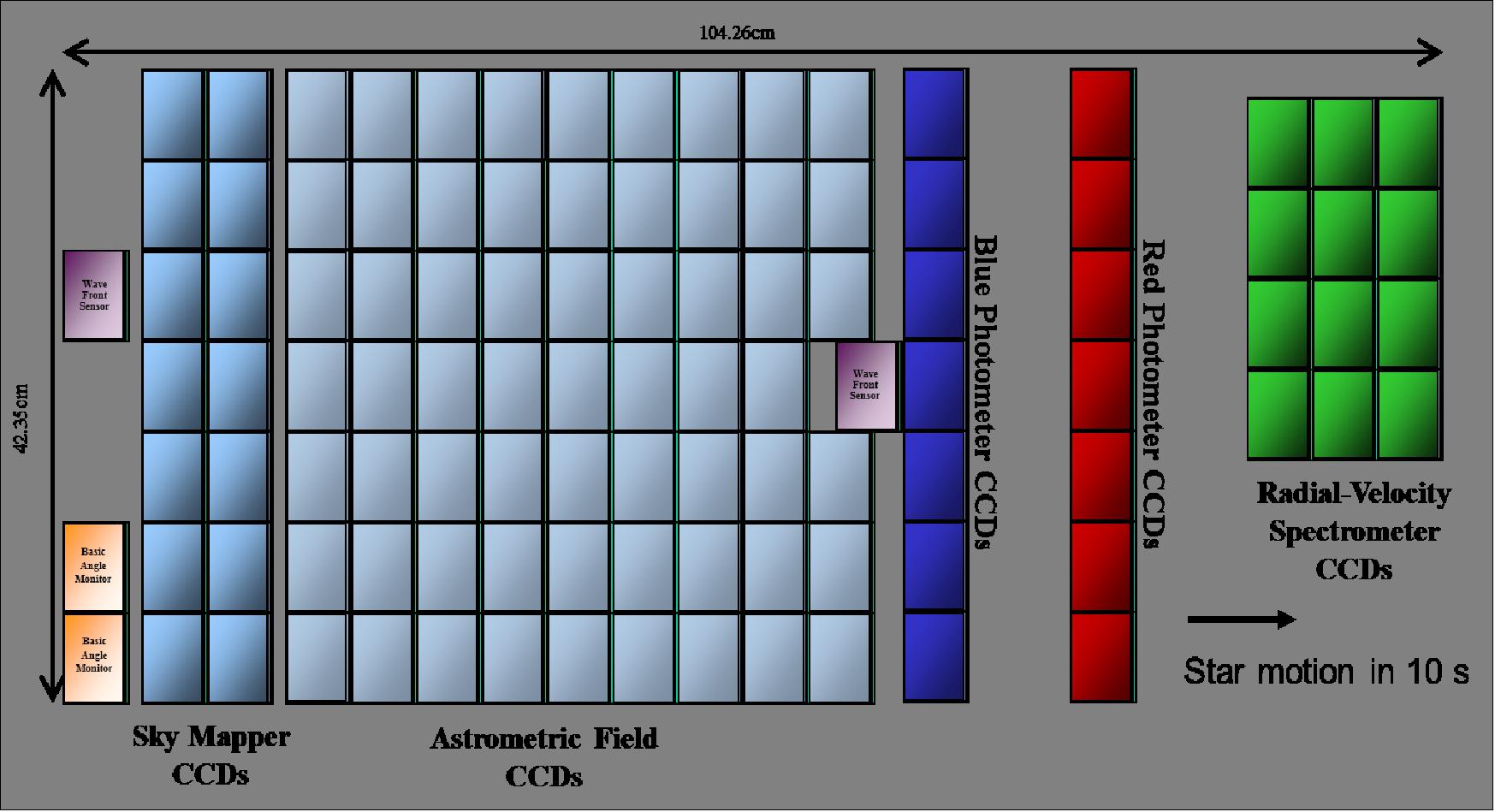
Function | No of CCDs | Details |
Metrology and alignment | 4 | 2 WFS (Wave Front Sensors), |
Star detection | 14 | 2 x 7 SM (Sky Mapper) CCDs, 7 per telescope |
Astrometry, AF (Astrometric Field) | 62 | 8 x 7, 1 x 6 |
Photometry (BP, RP) | 14 | 7 blue and 7 red |
Spectrometry (RVS) | 12 | 3 x 4 |
Observation Sequence in the Focal Plane: All CCDs, except those in the SM (Sky Mapper), are operated in windowing mode: only those parts of the CCD data stream, which contain objects of interest, are read out; remaining pixel data is flushed at high speed. The use of windowing mode reduces the readout noise to a handful of electrons while still allowing reading up to 20 objects simultaneously.
- Every object, crossing the focal plane, is first detected either by SM1 or SM2. These CCDs record, respectively, the objects only from telescope 1 or from telescope 2. This is achieved by a physical mask that is placed in each telescope's intermediate image, at M4/M41 beam-combiner level.
- Next,a surrounding window is allocated to the object, which is propagated through the following CCDs of the CCD row as the imaged object crosses the focal plane; the actual propagation uses input from the spacecraft's attitude control system, which provides the predicted position of each object in the focal plane versus time. After detection in SM, each object is confirmed by the CCD detectors in the first strip of the AF1 (Astrometric Field); this step eliminates false detections such as cosmic rays.
- The object then progressively crosses the eight next CCD strips in AF, followed by the BP, RP, and RVS detectors (the latter ones are present only for four of the seven CCD rows).
- The nominal integration time per CCD is 4.42 seconds, corresponding to 4500 pixels along scan. For bright saturating objects, the integration time in AF1-AF9, BP and RP is reduced by activating electronic TDI gates in the detector over a short period corresponding to the bright star window. The purpose of the TDI gates is to lower the effective number of pixels along scan. Twelve gates are available in the detector and allow optimizing the signal collection for bright stars at the minimum expense for faint stars.
CCD characteristics: All CCDs have the same format and are derived from e2V Technologies (UK) design and are large-area, back-illuminated, full-frame devices. They are operated in TDI (Time Delay Integration) mode with a TDI period of 982.8 µs. The focal plane is passively cooled to 170 K for reducing its sensitivity to radiation. The box shaped CCD radiator provides the radiative surface with the colder internal payload cavity (120 K) as well as CCD shielding against radiation and support for the photometer prisms.
The Gaia CCDs are fabricated in three variants, AF-, BP-, and RP-type, to optimize quantum efficiency corresponding to the different wavelength ranges of the scientific functions. The AF-type variant is built on standard silicon with broadband anti-reflection coating. It is the most abundant type in the focal plane, used for all but the photometric and spectroscopic functions. The BP-type only differs from the AF-type through the blue-enhanced backside treatment and anti-reflection coating, and it is exclusively used in BP. The RP-type is built on high-resistivity silicon with red-optimized anti-reflection coating to improve near-infrared response. It is used in RP as well as in RVS.
Pixel size along scan | 10 µm |
Pixel size across scan | 30 µm |
No of pixels along scan | 4500 |
No of pixels across scan | 1966 |
Total number of pixels/CCD | 8.847 Mpixels |
Total number of pixels in FPA with 106 CCDs | 937 Mpixels (or ~ 1 Gpixel), covering an area of about 0.38 m2 |
Operational temperature | -115ºC |

Payload module data handling 90)
Time reference: The star localization measurement is performed by transit detection and time measurement, which calls for a very accurate datation of object transits. For this purpose, a CDU (Clock Distribution Unit) provides all necessary timing signals and clock functions for video sample time tagging and ground based time scale correlation. All signals are generated on the basis of the highly stable central master 10 MHz Rubidium atomic clock.
Time correlation: In addition to the classical corrected one-way path technique, it is proposed that on-board-to-UTC time correlation be performed by a specific two way process. This technique allows to cancel symmetrical delays of ionospheric or tropospheric origin, or delays of relativistic origin. This correlation performance is furthermore independent of the orbit.
On-board Payload Processing: The PDHS (Payload Data Handling System) is implemented as a set of 7 VPUs (Video Processing Units), one for each detector row of the focal plane, feeding a common 960 GB solid state mass memory at PDHU. The processing part of the PDHS has a modular architecture which follows the FPA architecture and eases the accommodation. In the case of failure of one channel, this would have little impact on the science performance. The file-organized mass memory is a standard stand-alone unit.
Although Gaia has the biggest camera that has ever flown in space, Gaia does not actually take pictures in the conventional sense. Instead, it rather tracks the stars across its sensors as the telescopes rotate and the field of view moves across the star-filled sky. In order to do so, a constant readout of the onboard CCDs is done, and this takes a lot of computing power. For this, the seven high performance VPUs (Video Processing Units) are used which interface with the 'camera'. A VPU incorporates a dedicated Astrium-developed pre-processing board, and for the bulk of the processing, a SCS750 PowerPC board from Maxwell Technologies, Inc., of San Diego, USA. Each of the VPUs exhibits a processing power of more than 1000 MIPS (>1 GIPS). A VPU has a mass of 3.2 kg and a size of 195 x 120 x 253 mm. 91) 92) 93)
On-board data processing algorithms allow computations to be made in real time, without data buffering. The hardware-software share offers full flexibility and algorithms may be modified in-flight following first in-orbit results.
A precise centroiding of bright stars is made with the measurements performed in the first 2 columns of detectors in order to determine the star velocities for monitoring the spacecraft attitude control.


Metrology and alignment: Gaia embarks two specific devices, for recovery of the optical quality after launch and for continuous monitoring of the angle between the two telescope lines of sight. All these devices are operated at 130 K.
1) A five-degree-of-freedom static mechanism is implemented behind the secondary mirrors of the two telescopes , based on the TMA (Three Mirror Anastigmat) design, for securing the optical performance in orbit and cancelling static residual errors due to mirror micro-settings and gravity release. The BAM (Basic Angle Monitoring) system consists of a Fizeau interferometer, measuring fluctuations in the basic angle between the two telescopes. The image quality is measured by two Shark-Hartmann Wave Front Sensors using two dedicated CCDs in the focal plane. The signal is coming from bright stars.
2) During science operations, a Fizeau laser interferometer measures the in orbit fluctuation of the basic angle between the two input telescopes with an accuracy better than 0.5 µas. The fringe motion with respect to the detector frame provides the line-of-sight of the telescopes along scan, and therefore the basic angle variations.
Ground Segment
ESA's most powerful ground stations, the 35 m deep-space stations in New Norcia, Australia (DSA 1), Cebreros, Spain (DSA 2), and Malargüe, Argentina (DSA 3), will be used to send commands to Gaia and receive the high volume of science data that must be returned to Earth to create Gaia's Galactic Map. During the critical LEOP phase, additional ground station support will be provided by ESA's 15 m diameter Kourou, Maspalomas and Perth stations. 94) 95)
Communications and Orbit Tracking
• The end-to-end timing of the measurements must be highly precise. To this end, Gaia carries an atomic clock to time stamp the science data, which has to be matched by ultra-precise time stamping on ground at data reception. In fact, the CCSDS ground time-stamping standard that the space community uses was extended to a picosecond (10-12 s) resolution to meet the Gaia requirements, and this capability was added to ESA's Estrack Deep Space Antennas.
• The orbit of Gaia must also be determined to very high accuracy (to within 150 m at 1.5 million km). The traditional radiometric methods are supplemented by optical observations from ground-based telescopes, which take pictures of Gaia against the background stars, and ΔDOR (delta-Differential One-way Ranging) measurements in the commissioning phase (a method where multiple DSA stations are used to precisely determine the spacecraft position with respect to a Quasar).
• GBOT (Ground Based Orbit Tracking) campaign: GBOT utilizes a network of small-to-medium telescopes aiming to track the Gaia observatory. GBOT is committed to deliver one set of data per day, which allows the determination of Gaia's position good to 20 m arcsec. The GBOT data on Gaia will be included in the orbit reconstruction performed at ESOC in order to increase the accuracy of this undertaking to a level of 150 m in position and 2.5 mm/s in motion. These tight constraints are needed, to ensure that Gaia's measurements of the stars and Solar System objects are as accurate as possible. 96)
Astronomers within the DPAC community – first set up the GBOT project in early 2008 and trialled it on missions already in the same orbital location that Gaia will operate from – L2 – including NASA's WMAP and ESA's Planck satellites. This allowed the project to test their methods, and also get some clues about the probable magnitude that Gaia will have once in orbit. It is assumed that it will be around magnitude 18, but that it is still a big unknown.
Since then, a whole infrastructure was set up, developing observing techniques, a dedicated software pipeline, a database, and observatories were recruited to deliver the GBOT project data. The backbone of the data will be supplied by the 2 m Liverpool telescope, located on La Palma, Canary Islands, Spain, and the Las Cumbres Optical Global Telescope Network (LCOGT.net), which operates 1 m telescopes in Chile, South Africa, Australia and Texas. The project will also have some support from ESO's VST (2.6 m telescope at Paranal, Chile) and additional facilities will also provide data when needed.
In 2012, the project started a new fork of GBOT, radio-GBOT, which involves VLBI observations of Gaia. These are much more precise than the optical observations, but because they use more resources, the project will use this technique less often and therefore the radio data will be used only to complement the optical measurements.
The coordination of the GBOT activities is done from Heidelberg. The data reduction, analysis and storage, will be done at the Observatoire de Paris (with a mirror of the database in Heidelberg). The pipeline software, which has been developed by the GBOT group in Paris, imports and harmonizes the data obtained from the partner observatories, processes the data and finally outputs the position of Gaia. The data is then delivered to ESA's MOC (Mission Operations Center) in Darmstadt via the SOC (Science Operations Center) in Villafranca near Madrid. Likewise, the reconstructed orbit files from ESOC are retrieved by GBOT, converted into data on Gaia's position, with finder charts, and then supplied to the partner observatories.

Now, shortly before the launch of Gaia, GBOT is ready for action. GBOT's observations commence about 10 days after launch; any earlier and Gaia is too bright for the instruments of the partner institutes. The project hopes to obtain motion clips of the spacecraft moving in front of star fields as the satellite journeys towards L2. It will be a challenging task for the small team, but we will do our very best to deliver!
MSC (Mission Control System)
Mission operations will be conducted by the Flight Control Team at ESOC (European Space Operations Center) in Darmstadt Germany, comprising spacecraft operations (mission planning, spacecraft monitoring and control, and all orbit and attitude determination and control) as well as scientific instrument operations (quality control and collection of the science telemetry). The ground segment at ESOC will comprise all facilities, hardware, software and documentation required to conduct mission operations.
The ground operations facilities consist of:
• Ground stations and the communications network
• Mission control center
• FCS (Flight Control System)
• Software-based spacecraft simulator
All mission and flight control facilities, except the ground stations, are located at ESOC, including the interfaces for the provision of science telemetry to the SOC (Science Operations Center) at ESA/ESAC (European Space Astronomy Center), ESA facility in Villafranca, Spain, located about 30 km west of Madrid. 97)
The science data will be distributed to ESAC after being stored in dedicated Science Data Servers at ESOC, via high-speed communication lines.

The science data processing requirements for Gaia are among the most challenging of any scientific endeavor to date. Due to the immense volume of data that will be collected, for 1 billion stars, it will be a major challenge, even by the standards of computational power in the next decade, to process, manage and extract the scientific results necessary to build a 3-dimensional view of our Galaxy, the Milky Way.
A total of some 100 TB of science data will be collected during Gaia's lifetime. The estimated total data archive will surpass 1 PB (Petabyte or 1015 bytes), roughly equivalent to 1000 1 TB hard drives from a top-end home PC.
DPAC (Data Processing & Analysis Consortium)
Unlike a mission such as the Hubble Space Telescope, Gaia does not produce data that is immediately scientifically useful. The raw telemetry must first be processed before the sought after distances can be obtained, motions, and properties of the stars observed by Gaia. This immense task will be undertaken by a pan-European collaboration, the Gaia DPAC (Data Processing and Analysis Consortium). DPAC is responsible for the processing of Gaia‘s data with the final objective of producing the Gaia Catalogue. Drawing its membership from over 20 countries (Figure 93), the consortium brings together skills and expertise from across the continent, reflecting the international nature and cooperative spirit of ESA itself.
The DPAC consists of about 450 persons, spread over academic institutes and space agencies throughout Europe and beyond, who are actively contributing to writing the millions of lines of code needed for the data processing and to subsequently operate the software systems and validate the resulting output. Each DPAC is responsible for a different aspect of the Gaia data processing. 98) 99) 100) 101)

Legend to Figure 93: Next to the European country DPACs, there are also members in Brazil, Canada, Chile, Israel, and the USA.
To organize the large amount of tasks to be carried out, the DPAC has been subdivided into nine specialist units known as CUs (Coordination Units). Each CU takes the responsibility for the development of a specific part of the Gaia data processing: system architecture, simulations, astrometry, photometry, spectroscopy, object processing, variability processing, astrophysical parameters, and catalog publication. The CUs draw their membership from multiple countries.

The astronomers in the CUs conceive the scientific algorithms for the data processing and also carry out a large fraction of the software development. The software is then run at one of the six DPCs (Data Processing Centers). The personnel at the data processing centers also provide the much needed software engineering expertise. Such a large software system cannot be developed and operated by astronomers alone!
The schematic of Figure 94 shows how each CU is supported by a specific DPC (indicated in red). The data exchange within DPAC will take place through the so-called MBD (Main Data Base), housed at ESAC. After the completion of a processing cycle, data is then extracted from the MDB and prepared for release.
Note that the Gaia project is unique in that the scientific data produced by DPAC are not subject to a proprietary period. On completion of a processing cycle the results are immediately available to the scientific community and also to the general public. The nine CUs and six DPCs are coordinated by an executive committee, the DPACE (DPAC Executive) as shown in Figure 95. 102)

Gaia's VO (Virtual Observatory) Big Data Archive
ESA's Gaia mission will survey the sky for at least 5 years providing high accuracy astrometry, radial velocities and multi-color photometry. The DPAC (Data Analysis and Processing Consortium) efforts will result in an astronomical catalog with unprecedented accuracy and completeness of at least 1 billion (109) sources, and over 1PB of associated data products. 103)
This brings big data challenges in storing, querying and distributing all the associated data and meta data, comparing them with other astronomical catalogues, enabling analysis, visualization, data mining and then sharing these results with other scientists. The amount of data involved forces a change of paradigm in dealing with astronomy archives. The usual usage of downloading the data to the users for her/him to work further on it needs towards evolve to a new way of working where the users' can send her/his code to the data, run it there on computing and storage services provided directly by the archive, where the data reside. The Gaia archive will provide an infrastructure to run added value interfaces and software on top of the Gaia data.
Nominal mission data up to January 2016 — with associated products up to 1 PB pf Gaia data by mission end | ||
Type of data | Amount (Byte) | Description |
Science telemetry | 28 TB |
|
Astronomy transits | 35.2 x 109 | 352.3 x 109 measurements |
Photometry transits | 35.2 x 109 | 73.6 x 109 low resolution spectra |
Spectroscopy transits | 2.3 x 109 | 6.9 x 109 high resolution spectra |
Main database | 90 TB | 700 TB expected after 5 years |
The first Gaia catalog will be publicly released to the scientific community around summer 2016, but the development of the Gaia archive has long started and an internal version is already available for the Gaia Consortium.
The term Big Data can be used and understood differently by people, but today industry widely refers to the five "Vs" (Volume, Velocity, Variety, Veracity and Value) when speaking about Big Data. From the Volume perspective, with "only" 1 PB of data produced by the end of its lifetime, Gaia could hardly be considered as a big data mission, as many other astronomy projects (in particular ground based telescope) will produce way more data volume in a shorter timescale.
The final delivery will also include all the single epoch CCD transit data that was used in the catalog computation, reaching an estimate of around 1PB at the end of the mission in 2022 (Table 5).
Nonetheless, the billions of CCD transits, measurements and spectra (Table 5) that will result into massive sources catalogs (Figure 96) for sure makes Gaia a big data challenge, from the data Velocity and Variety points of view.

Ensuring the Veracity of the Gaia data represents one of the main big data challenge of the Gaia data processing. And to finish, by performing astrometry, photometry and spectroscopy of about one billion objects in our Milky Way galaxy and beyond, the extent and content of the Gaia Catalogs will enable major progress to be made in many fields of galactic and stellar astronomy, hence its Value definitely places Gaia as a major Big Data project in astronomy.
Standard Archive Architecture
Standard ESA space science archive architecture (Figure 3) is based on the OAIS (Open Archival Information System) architecture. Users and scientists are used to interact with data or catalogues, either through a browser user interface or scriptable command line interface. They download the data and the full catalogue, usually via FTP, to their local disk and perform their science analysis on their local computer.
This working model works fine for small amount of data, but becomes difficult as data volume grows. To reduce the data transfer burden, the users try to select region of the sky and download only part of the catalogue, and then combine it with their own datasets or catalogues already stored on their disk. This can be described as "move the data to the computing facility".

The VO (Virtual Observatory) provides an unified framework which enables transparent access to astronomical science data holdings coming from various different archives. The very same command can be sent to archives located in different locations (and using their own internal storage and database systems) and present results in a consistent way to the end user or applications. Early developed interoperability VO protocols (e.g., ConeSearch, Simple Image and Simple Spectra Access) greatly facilitates access to multiple datasets, but still assumes that data are finally being downloaded to the user's computer.
Usually, science archives implements a "VO layer" on top of the existing archive infrastructure, so all the data holdings can be accessible through these VO protocols. This ensures the interoperability of the archives with other VO compliant archives and applications.
New Archive Paradigm for Gaia
With the avalanche of data in astronomy, the archive model previously described reaches its limit and a new paradigm needs to be established, the so called "move the code to the data". For Gaia, the amount of data and meta data is so big that special computing infrastructure is required to efficiently handle Gaia data. For example, a query of a cone search on the ~1 billion Gaia catalog might return 10 million sources. Another typical use case is to upload a table with sources and to cross match these with the Gaia catalog. This operation is made possible with the VO TAP (Table Access Protocol), coupled with ADQL (Astronomical Data Query Language, SQL with specialized astronomical searches). TAP also supports asynchronous query, as such a cross match can require too much time to be perform interactively. UWS (Universal Worker Service) enters in action to manage these asynchronous jobs. This back-end infrastructure serves all the front-end interfaces available at the Gaia archive.
Special emphasis has been put on the database design (based on PostgreSQL and pgSphere add-on which provides spherical data types, functions, and operators for PostgreSQL) and associated indexing. Furthermore, the Gaia archive is hosted on a powerful server and the most popular catalogs are stored on PCIe SSDs disks to ensure good performance of these crossmatch functions. Some examples of time required for some of the complex crossmatches are given in Table 6.
Note: PCIe (Peripheral Component Interconnect Express); SSD (Solid State Drive).
Catalog 1 | Catalog 2 | Radius (arcsec) | # results | Time |
Tycho2 | 2 MASS PSC | 1" | 2,495,304 | 49 s |
Tycho2 | 2 MASS PSC | 5" | 2,614,163 | 116 s |
Tycho2 | IGSL | 1" | 2,600,542 | 46 s |
Tycho2 | IGSL | 5" | 2,829,401 | 55 s |
Legend to Table 6: Tycho2 vs. IGSL (Initial Gaia Source List) crossmatches are even faster than the ones with 2MASS as IGSL is located in the fastest local storage (PCIe), even when IGSL (similar to the final Gaia catalog) is around 3 times bigger than 2MASS.
End user can then interact with the Gaia through a standard GUI (Graphical User Interface) from any standard web browser. This offers a full ADQL query interface, with example to help the user familiarize with the Gaia catalog content and structure.
In addition, it is expected that many of the users will interface with Gaia data directly through scriptable interface. All operations available from the Gaia archive GUI (ie TAP/ADQL queries) can also be included directly in user's scripts. Various examples of such scripts are provided in the most commonly used programming language in astronomy (Python, C, Java).
VO applications (e.g., Topcat, VOSpec) can access directly the Gaia data through the corresponding VO protocol, TAP for table, Simple Access Protocol for spectra, without the need to develop a "VO layer" as seen in the standard ESA archive architecture.
When the user retrieves big volume of Gaia data, she/he would be able to download it to her/his local disk via FTP, but it will probably be more efficient to leave it on the archive disk itself to avoid the burden of the network transfer. This can be done with VOSpace, virtual disk accessible by VO data access protocols. By keeping the data on her/his VOSpace, the user can continue to interact with it and as well share it with any other Gaia archive user, to facilitate scientific collaboration.

A similar mechanism is provided for meta data storage. If the user would download the results of a complex search (i.e., returning millions of entries) on her/his computer, she/he will probably need to ingest these into a local database to continue to work on them. It appears then more efficient to provide to the user some space directly within the Gaia archive database to store the results of her/his searches. As such, the user "database" becomes VO compliant and shareable as well with any other Gaia archive user.
Overall, the Gaia archive architecture depicted in Figure 98 has been built around these VO protocols and has become the first "VO built-in" archive. By design, the Gaia archive also becomes immediately interoperable with any other VO compliant archive and application.
Gaia AVIs (Added Value Interfaces)
The user workspace can be brought one step further to enable the user to also run her/his own code directly on the Gaia archive through so called "Gaia Added Value Interfaces" (Gaia AVIs, Figure 99). Four AVI demonstrators are currently being developed for transient alerts, advanced visualization, spectral classification and temporal analysis. These AVIs will run using containers (Docker) and will make use of the Gaia Archive VO built-in protocols (TAP, VOSpace). A Gaia AVI Portal will be created and users will be able deposit their own code and can run it on their data located into their user workspace (VOSpace and database). AVI templates will be provided to help the user to develop their own AVIs.
The Gaia AVI project is currently being developed as a proof of concept project to be delivered in 2017 and could become the new framework for collaborative "Archive 2.0".

Big Data Visualization
Before being able to search for Gaia data, it might be really helpful to provide visualization of the Gaia data in various ways, such as density maps, 1D histograms (Figure 100) or again interactive visualization through VO application (Aladin Lite), integrated into the archive GUI, through another VO protocol SAMP (Simple Application Messaging Protocol). The production of such graphs requires the use of big data reduction techniques, such as Map / Reduce. With 10 parallel threads on a powerful machine with big RAM (1TB), fast disks (PCIe SSDs with fast random IO) and efficient database (PostgreSQL), the production of the density maps for the GUMS simulated catalogue (2.14 billion rows) took less than 2 minutes and the ones for the IGSL (1.22 billion rows) as little as 65 seconds.

Another way to visualize Gaia data will be through the recently released science-driven discovery portal for all the ESA Astronomy Missions called "ESA Sky" that allow users to explore the multi-wavelength sky and to seamlessly retrieve science-ready data in all ESA Astronomy mission archives from a web application without prior-knowledge of any of the missions. Among other things, the system offers progressive multi-resolution all-sky projections of full mission datasets using a new generation of HiPS (Hierarchical Progressive Survey) files. HiPS is based on the HEALPix sky tessellation and is essentially a mapping of survey data at various spatial resolutions into a collection of HEALPix tiles. It is particular adapted to big data visualization as it allows a dedicated client/browser tool to access and display a survey progressively, based on the principle that "the more you zoom in on a particular area the more details show up".
Summary: Gaia, ESA's cornerstone mission currently in operations, represents one of the major big data challenge in astronomy to date. A totally new archive architecture (both hardware and software) has been developed to tackle this challenge. It results into one of the first VO built-in science archive, paving the way towards flexible, open and interoperable archive services. The user will work directly with the data in the archive through dedicated user workspace, without the need to transfer it to her/his location, She/he will be able to become an actor of the archive with the possibility to bring her/his code to the data and share it with other archive users. This new "Archive 2.0" concept will be the mean to fully exploit the science legacy of the Gaia mission.
References
1) "Gaia Overview," ESA, June 19, 2013, URL: http://www.esa.int/Our_Activities/Space_Science/Gaia_overview
2) S. Clark, "Gaia - ESA's Galactic Census," ESA brochure, BR-296, June 2012, URL: http://sci.esa.int/gaia/50670-gaia-esa-s-galactic-census-esa-br-296/#
3) M.A.C. Perryman, O. Pace, "Gaia – Unravelling the Origin and Evolution of Our Galaxy," ESA Bulletin 103, August 2000, URL: http://www.esa.int/esapub/bulletin/bullet103/perryman103.pdf
4) Philippe Gare, Giuseppe Sarri, Rudolf Schmidt, "ESA's 'Billion-Pixel' Camera - The challenges of the Gaia mission," ESA Bulletin, No 137, February 2009, pp.50-59, URL: http://www.esa.int/esapub/bulletin/bulletin137/bul137g_sarri.pdf
5) "Gaia - Science with 1 billion objects in three dimensions," ESA, URL: http://www.cosmos.esa.int/web/gaia/home
6) Eric Maliet, Philippe Charvet, François Chassat, Giuseppe Sarri, Robert Furnell, Timo Prusti, "Gaia spacecraft in-orbit commissioning status," Proceedings of the 65th International Astronautical Congress (IAC 2014), Toronto, Canada, Sept. 29-Oct. 3, 2014, paper: IAC-14.A7.1.4
7) Note: The Hipparcos mission is named after the ancient Greek astronomer Hipparchus who, in 129 BC and using only naked eye observations and simple geometry, catalogued the relative positions of around one thousand stars.
8) M. McCaughrean (editor), "ESA's Report to the 39th COSPAR Meeting," (ESA SP-1323, June 2012), pp. 321-324, URL: http://sci.esa.int/home/50671-esa-report-to-the-39th-cospar-meeting-esa-sp-1323/#
9) "Guide to our Galaxy," ESA, Nov. 21, 2013, URL: http://spaceinvideos.esa.int/Videos/2013/11/Guide_to_our_Galaxy
10) L. Lindegren, M. A. C. Perryman, "GAIA Global Astrometric Interferometer for Astrophysics, A Concept for an ESA Cornerstone Mission," 1995, URL: http://gaia.obspm.fr/IMG/pdf/Lindegren_Perryman_1995.pdf
11) "Gaia launch postponement update," ESA, Oct. 23, 2013, URL: http://www.esa.int/Our_Activities/Space_Science/Gaia/Gaia_launch_postponement_update
12) Devin Powell, "Europe's Star Power," Nature, Vol. 502, October 3, 2013, URL: http://www.nature.com/polopoly_fs/1.13859!/menu/main/topColumns/topLeftColumn/pdf/502022a.pdf
13) "Gaia Fact Sheet," ESA, June 19, 2013, URL: http://www.esa.int/Our_Activities/Space_Science/Gaia/Gaia_factsheet
14) "Spacecraft development," EADS Astrium, Nov. 2007, URL: http://www.astro.lu.se/ELSA/pages/PublicDocuments/Moisson.pdf
15) S. Clark, K. Fletcher, "Gaia - ESA's Galactic Census," ESA brochure, Vol. BR-296, June 15, 2012, URL: http://sci.esa.int/gaia/50670-gaia-esa-s-galactic-census-esa-br-296/#
16) https://web.archive.org/web/20150116183538/http://www.space-airbusds.com/media/document/gaia-data-sheet.pdf
17) "Industrial involvement in the Gaia spacecraft," ESA, URL: http://sci.esa.int/gaia/49708-industrial-team/
18) " .....In the world of Gaia," URL: http://hvossgaia.wordpress.com/
19) Patrick Blau, "Gaia Spacecraft Overview," Spaceflight 101, URL: http://www.spaceflight101.com/gaia-spacecraft-overview.html
20) "The Gaia Spacecraft and Instruments," ESA, URL: http://www.rssd.esa.int/SA/GAIA/docs/posters/InstrumentsNoAuthor.pdf
21) Giuseppe Sarri, Timo Prusti, Ared Schnorhk, "ESA's Billion-Star Surveyor: Gaia Ready for Launch Campaign," ESA Bulletin 155, August 2013, pp. 2-11, URL: http://esamultimedia.esa.int/multimedia/publications/ESA-Bulletin-155/
22) "Service Module," ESA, June 14, 2013, URL: http://www.esa.int/Our_Activities/Space_Science/Gaia/Service_module
23) "Service Module Summary," ESA, URL: http://sci.esa.int/gaia/40130-service-module/?fbodylongid=1910
24) "Gaia mission summary," ESA, URL: http://sci.esa.int/gaia/28820-summary/
25) "Gaia Mission - General Overview," ESA, April 13, 2015, URL: http://www.sscspace.com/gaia-mission-general-overview
26) "Gaia Flight Model Payload Data Handling Unit Delivered and Integrated," ESA, May 23, 2013, URL: http://sci.esa.int/gaia/51777-09-gaia-flight-model-payload-data-handling-unit-delivered-and-integrated/
27) Christophe Delay, Stephane Humbert, "1 Tbit of data serviced by SpW," Proceedings of the 5thInternational SpaceWire Conference 2013, Gothenburg, Sweden, June 10 - 14, 2013, pp: 167-170, URL: http://2013.spacewire-conference.org/downloads/SpW2013ProceedingsFinal.pdf
28) A. Polli, G. Noci, L. Ceruti, L. Fallerini, A. Atzei, "European Cold Gas Micro Propulsion System reached TRL-9 on board Gaia S/C. Discussion on in flight performance and system flexibly and capabilities, for being implemented in other missions requiring ultra-fine satellite positioning and attitude control," Proceedings of the 65th International Astronautical Congress (IAC 2014), Toronto, Canada, Sept. 29-Oct. 3, 2014, paper: IAC-14,C4,3.3
29) Raffaele Vitulli, Enrico Magli, Sean Blair, "Putting the squeeze on : How data compression can stop space missions drowning in data," ESA Bulletin, No 158, May 2014, pp: 48-59, URL: http://www.esa.int/About_Us/ESA_Publications/ESA_Bulletin_158_May_2014
30) "GTD's successful delivery of the product named GOCA to ESA/ESTEC," Feb. 26, 2008, URL: http://www.gtd.eu/fr/news-and-events/gtds-successful-delivery-product-named-goca-esaestec
31) Information provided by Jordi Portell i de Mora - Gaia Team at IEEC-UB Dept. Física Quàntica i Astrofísica, Universitat de Barcelona, Spain
32) "IEEC post on GOCA," URL: http://www.ieec.cat/project/gaia/
33) "Gaia Data Release 1 (DR1)," ESA and Gaia Data Processing and Analysis Consortium, Sept. 20, 2016, URL: http://gaia.esac.esa.int/documentation/GDR1/pdf/GaiaDR1_documentation_D.0.pdf ( see Video Processing Unit and Algorithms on p. 23)
34) "Gaia secured inside fairing," ESA, Dec. 13, 2013, URL: http://www.esa.int/Our_Activities/Space_Science/Gaia/Gaia_secured_inside_fairing
35) "Liftoff for ESA's billion-star surveyor," ESA PR 44-2013, December 19, 2013, URL: http://sci.esa.int/gaia/53536-esa-pr-44-2013-liftoff-for-esas-billion-star-surveyor/
36) "Next stop ..... the stars! Arianespace successfully lofts Gaia on Soyuz," Arianespace, Decmber 19, 2013, Soyuz Flight VS06, URL: http://www.arianespace.com/news-mission-update/2013/1120.asp
37) Giuseppe Sarri, Timo Prusti and Ared Schnorhk, "ESA's Billion-Star Surveyor, Gaia ready for launch campaign," ESA Bulletin, No 155, August 2013, pp. 2-11
38) "Gaia data release 3: exploring our multi-dimensional Milky Way," ESA Science & Exploration, 13 June 2022, URL: https://www.esa.int/ESA_Multimedia/Videos/2022/06/Gaia_data_release_3_exploring_our_multi-dimensional_Milky_Way
39) "Gaia sees strange stars in most detailed Milky Way survey to date," ESA Science & Exploration, 13 June 2022, URL: https://www.esa.int/Science_Exploration/Space_Science/Gaia/Gaia_sees_strange_stars_in_most_detailed_Milky_Way_survey_to_date
40) "Gaia – ESA's billion star surveyer," ESA Science & Exploration, 13 May 2022, URL: https://www.esa.int/ESA_Multimedia/Videos/2022/05/Gaia_ESA_s_billion_star_surveyer
41) "Gaia finds parts of the Milky Way much older than expected," ESA Science & Exploration, 23 March 2022, URL: https://www.esa.int/Science_Exploration/Space_Science/Gaia/Gaia_finds_parts_of_the_Milky_Way_much_older_than_expected
42) Maosheng Xiang & Hans-Walter Rix, "A time-resolved picture of our Milky Way's early formation history," Nature, Volume 603, pp: 599-603, Published: 23 March 2022, https://doi.org/10.1038/s41586-022-04496-5, URL: https://www.nature.com/articles/s41586-022-04496-5.pdf
43) "Gaia snaps photo of Webb at L2," ESA Science & Exploration, 16 March 2022, URL: https://www.esa.int/Science_Exploration/Space_Science/Gaia/Gaia_snaps_photo_of_Webb_at_L2
44) "Gaia reveals a new member of the Milky Way family," ESA Science & Exploration, 17 February 2022, URL: https://www.esa.int/ESA_Multimedia/Images/2022/02/Gaia_reveals_a_new_member_of_the_Milky_Way_family
45) Khyati Malhan, Rodrigo A. Ibata, Sanjib Sharma, Benoit Famaey, Michele Bellazzini, Raymond G. Carlberg, Richard D'Souza, Zhen Yuan, Nicolas F. Martin, and Guillaume F. Thomas, "The Global Dynamical Atlas of the Milky Way Mergers: Constraints from Gaia EDR3–based Orbits of Globular Clusters, Stellar Streams, and Satellite Galaxies," The Astrophysical Journal, Volume 926, Number 2, Published: 17 February 2022, https://doi.org/10.3847/1538-4357/ac4d2a, URL: https://iopscience.iop.org/article/10.3847/1538-4357/ac4d2a/pdf
46) "Colliding star clusters," Physics Today, 3 January 2022, URL: https://physicstoday.scitation.org/do/10.1063/PT.6.1.20220103a/full/
47) Andrés E Piatti, Khyati Malhan, "First evidence of a collision between two unrelated open clusters in the Milky Way," Monthly Notices of the Royal Astronomical Society: Letters, slab130, Published: 13 December 2021, https://doi.org/10.1093/mnrasl/slab130
48) "Texas Astronomers Discover Strangely Massive Black Hole in Milky Way Satellite Galaxy," UT News, 1 December 2021, URL: https://news.utexas.edu/2021/12/01/texas-astronomers-discover-strangely-massive-black-hole-in-milky-way-satellite-galaxy/
49) M. J. Bustamante-Rosell, Eva Noyola, Karl Gebhardt, Maximilian H. Fabricius, Ximena Mazzalay, Jens Thomas, and Greg Zeimann, "Dynamical Analysis of the Dark Matter and Central Black Hole Mass in the Dwarf Spheroidal Leo I," The Astrophysical Journal, Volume 921, Number 2, Published: 5 November 2021, https://doi.org/10.3847/1538-4357/ac0c79
50) "Gaia reveals that most Milky Way companion galaxies are newcomers to our corner of space," ESA Science & Exploration, 24 November 2021, URL: https://www.esa.int/Science_Exploration/Space_Science/Gaia/Gaia_reveals_that_most_Milky_Way_companion_galaxies_are_newcomers_to_our_corner_of_space
51) Francois Hammer, Jianling Wang, Marcel S. Pawlowski, Yanbin Yang, Piercarlo Bonifacio, Hefan Li, Carine Babusiaux and Frederic Arenou,"Gaia EDR3 Proper Motions of Milky Way Dwarfs. II Velocities, Total Energy, and Angular Momentum," The Astrophysical Journal, Volume 922, Number 2, Published: 24 November 2021, https://doi.org/10.3847/1538-4357/ac27a8
52) "Stream of stars extends thousands of light-years across the Milky Way," Space Daily, 8 June 2021, URL: https://www.spacedaily.com/reports/Stream_of_stars_extends_thousands_of_light_years_across_the_Milky_Way_999.html
53) L. G. Bouma, J. L. Curtis, J. D. Hartman, J. N. Winn, & G. Á. Bakos, "Rotation and Lithium Confirmation of a 500 Parsec Halo for the Open Cluster NGC 2516," the paper has been submitted to the journals of the American Astronomical Society (AAS) and will be shared with the media at the 238th AAS meeting on Monday, June 7, 2021.
54) "Astronomers Release New All-Sky Map of Milky Way's Outer Reaches," NASA/JPL News, 21 April 2021, URL: https://www.jpl.nasa.gov/news/astronomers-release-new-all-sky-map-of-milky-ways-outer-reaches?utm_source=iContact&utm_medium=email&utm_campaign=nasajpl&utm_content=daily20210421-3
55) Charlie Conroy, Rohan P. Naidu, Nicolás Garavito-Camargo, Gurtina Besla, Dennis Zaritsky, Ana Bonaca & Benjamin D. Johnson, "All-sky dynamical response of the Galactic halo to the Large Magellanic Cloud," Nature, Volume 592, pp: 534-536, Published: 21 April 2021, https://doi.org/10.1038/s41586-021-03385-7
56) "Is the nearest star cluster to the Sun being destroyed?," ESA Science & Exploration, 24 March 2021, URL: https://www.esa.int/Science_Exploration/Space_Science/Gaia/Is_the_nearest_star_cluster_to_the_Sun_being_destroyed
57) Tereza Jerabkova, Henri M. J. Boffin, Giacomo Beccari, Guido de Marchi, Jos H. J. de Bruijne and Timo Prusti, "The 800 pc long tidal tails of the Hyades star cluster - Possible discovery of candidate epicyclic overdensities from an open star cluster," Astronomy & Astrophysics, Volume 647, Article No A137, Published online: 24 March 2021, https://doi.org/10.1051/0004-6361/202039949
58) "Gaia's new data takes us to the Milky Way's anticenter and beyond," ESA / Science & Exploration / Space Science / Gaia, 03 December 2020, URL: https://www.esa.int/Science_Exploration/Space_Science/Gaia/Gaia_s_new_data_takes_us_to_the_Milky_Way_s_anticentre_and_beyond
59) "Gaia Data Processing and Analysis Consortium," ESA Science & Exploration, 3 December 2020, URL: https://www.esa.int/ESA_Multimedia/Images/2020/12/Gaia_Data_Processing_and_Analysis_Consortium
60) "Star Clusters are only the tip of the iceberg," University of Vienna Press Release, 15 October 2020, URL: https://medienportal.univie.ac.at/presse/aktuelle-pressemeldungen/detailansicht/artikel/star-clusters-are-only-the-tip-of-the-iceberg/
61) Stefan Meingast, João Alves, and Alena Rottensteiner, "Extended stellar systems in the solar neighborhood, Discovery of coronae of nearby star clusters," Accepted for Publication in Astronomy & Astrophysics, manuscript as of October 2, 2020, URL: https://www.aanda.org/articles/aa/pdf/forth/aa38610-20.pdf
62) "Preliminary info on Gaia EDR3 contents," ESA, 14 July 2020, URL: https://www.cosmos.esa.int/web/gaia/news
63) "Gaia revolutionizes asteroid tracking," ESA Safety & Security, 1 July 2020, URL: https://www.esa.int/Safety_Security/Gaia_revolutionises_asteroid_tracking
64) "Chance of finding young Earth-like planets higher than previously thought, say Sheffield scientists," The University of Sheffield News, 5 June 2020, URL: https://www.sheffield.ac.uk/news/nr/finding-earth-like-planets-physics-astronomy-sheffield-1.890298
65) Amy L. Bottrill, Molly E. Haigh, Madeleine R. A. Hole, Sarah C. M. Theakston, Rosa B. Allen, Liam P. Grimmett, and Richard J. Parker, "Exoplanet Detection and Its Dependence on Stochastic Sampling of the Stellar Initial Mass Function," The Astrophysical Journal, Volume 895, Number 2, Published: 5 June 2020, https://doi.org/10.3847/1538-4357/ab8e39
66) "Unlocking clues to the origins of the stars," University of Leeds, Science News, 4 June 2020, URL: http://www.leeds.ac.uk/news/article/4602/unlocking_clues_to_the_origins_of_the_stars
67) M. Vioque, R. D. Oudmaijer, M. Schreiner, I. Mendigutía, D. Baines, N. Mowlavi and R. Pérez-Martínez, "Catalogue of new Herbig Ae/Be and classical Be stars," Astronomy & Astrophysics, Volume 638, 4 June 2020, https://doi.org/10.1051/0004-6361/202037731
68) "Galactic crash may have triggered Solar System formation," ESA / Science & Exploration / Space Science / Gaia, 25 May 2020, URL: http://www.esa.int/Science_Exploration/Space_Science/Gaia/Galactic_crash_may_have_triggered_Solar_System_formation
69) Chris W. Purcell, James S. Bullock, Erik J. Tollerud, Miguel Rocha & Sukanya Chakrabarti, "The Sagittarius impact as an architect of spirality and outer rings in the Milky Way," Nature, Volume 477, pp: 301-303, 14 September 2011, https://doi.org/10.1038/nature10417
70) Tomás Ruiz-Lara, Carme Gallart, Edouard J. Bernard & Santi Cassisi, "The recurrent impact of the Sagittarius dwarf on the star formation history of the Milky Way," Nature Astronomy, 25 May 2020, https://doi.org/10.1038/s41550-020-1097-0
71) "Gaia DR2 - second anniversary," Cosmos ESA, 25 April 2020, URL: https://www.cosmos.esa.int/web/gaia
72) "Gaia newsletter #10," Cosmos ESA, 20 March 2020, URL: https://www.cosmos.esa.int/web/gaia/newsletter/contents#GaiaNewsletter10
73) "Milky Way's warp caused by galactic collision, Gaia suggests," ESA / Science & Exploration / Space Science / Gaia, 2 March 2020, URL: http://www.esa.int/Science_Exploration/Space_Science/Gaia/Milky_Way_s_warp_caused_by_galactic_collision_Gaia_suggests
74) E. Poggio, R. Drimmel, R. Andrae, C. A. L. Bailer-Jones, M. Fouesneau, M. G. Lattanzi, R. L. Smart & A. Spagna, "Evidence of a dynamically evolving Galactic warp," Nature Astronomy Letter, Published 02 March 2020, https://doi.org/10.1038/s41550-020-1017-3
75) "Kazan University's telescope assists in discovering invisible stars," Kazan University, 13 February 2020, URL: https://kpfu.ru/eng/news-eng/microlensing-event-telescope.html
76) "RIT scientists discover the nearest-known ‘baby giant planet'," RIT (Rochester Institute of Technology), 10 February 2020, URL: https://www.rit.edu/science/news/rit-scientists-discover-nearest-known-baby-giant-planet
77) Global Gaia campaign reveals secrets of stellar pair," ESA / Science & Exploration / Space Science / Gaia, 21.January 2020, URL: http://www.esa.int/Science_Exploration/Space_Science/Gaia/Global_Gaia_campaign_reveals_secrets_of_stellar_pair
78) "Stellar pair discovered in Gaia16aye microlensing event," ESA Science & Exploration, 21 January 2020, URL: http://www.esa.int/Science_Exploration/Space_Science/Gaia/Global_Gaia_campaign_reveals_secrets_of_stellar_pair
79) Ł. Wyrzykowski, P. Mróz, K. A. Rybicki, M. Gromadzki, Z. Kołaczkowski, M. Zieliński, P. Zieliński, N. Britavskiy, A. Gomboc, K. Sokolovsky, S. T. Hodgkin, L. Abe, G. F. Aldi, A. AlMannaei, G. Altavilla, A. Al Qasim, G. C. Anupama, S. Awiphan, E. Bachelet, V. Bakış, S. Baker, S. Bartlett, P. Bendjoya, K. Benson, I. F. Bikmaev, G. Birenbaum, N. Blagorodnova, S. Blanco-Cuaresma, S. Boeva, A. Z. Bonanos, V. Bozza, D. M. Bramich, I. Bruni, R. A. Burenin, U. Burgaz, T. Butterley, H. E. Caines, D. B. Caton, S. Calchi Novati, J. M. Carrasco, A. Cassan, V. Čepas, M. Cropper, M. Chruślińska, G. Clementini, A. Clerici, D. Conti, M. Conti, S. Cross, F. Cusano, G. Damljanovic, A. Dapergolas, G. D'Ago, J. H. J. de Bruijne, M. Dennefeld, V. S. Dhillon, M. Dominik, J. Dziedzic, O. Erece, M. V. Eselevich, H. Esenoglu, L. Eyer, R. Figuera Jaimes, S. J. Fossey, A. I. Galeev, S. A. Grebenev, A. C. Gupta, A. G. Gutaev, N. Hallakoun, A. Hamanowicz, C. Han, B. Handzlik, J. B. Haislip, et al., "Full orbital solution for the binary system in the northern Galactic disc microlensing event Gaia16aye," Astronomy & Astrophysics, Volume 633, January 2020,Article Nr. A98, Published online: 21 January 2020, https://doi.org/10.1051/0004-6361/201935097
80) Mary Todd Bergman, "Interconnected stellar nurseries form the largest gaseous structure ever observed in the Milky Way galaxy," The Harvard Gazette, 7 January 2020, URL: https://enervated/gazette/story/2020/01/largest-gaseous-structure-ever-seen-in-our-galaxy-is-discovered/
81) João Alves, Catherine Zucker, Alyssa A. Goodman, Joshua S. Speagle, Stefan Meingast, Thomas Robitaille, Douglas P. Finkbeiner, Edward F. Schlafly & Gregory M. Green, "A Galactic-scale gas wave in the solar neighborhood," Nature, Published: 07 January 2020, https://doi.org/10.1038/s41586-019-1874-z
82) "Payload Module Summary," ESA, Oct. 12, 2013, URL: http://sci.esa.int/gaia/40129-payload-module/
83) "Spacecraft & Instruments," ESA, URL: http://www.cosmos.esa.int/web/gaia/spacecraft-instruments
84) "Payload module," ESA, URL: http://www.cosmos.esa.int/web/gaia/payload-module
85) Philippe Gare, Giuseppe Sarri, Rudolf Schmidt, "ESA's 'Billion-Pixel' Camera," ESA Bulletin 137, February 2009, URL: http://www.esa.int/esapub/bulletin/bulletin137/bul137g_sarri.pdf
86) Patrick Blau, "Gaia Instrument Information," Spaceflight 101, URL: http://www.spaceflight101.com/gaia-science-instruments.html
87) "Gaia Mission - General Overview," SSC, April 13, 2015, URL: http://www.sscspace.com/gaia-mission-general-overview
88) http://www.cosmos.esa.int/web/gaia/focal-plane
89) http://sci.esa.int/gaia/40129-payload-module/?fbodylongid=1907
90) http://sci.esa.int/gaia/40129-payload-module/?fobjectid=40129&fbodylongid=1909
91) "On the edge of the possible," Gaia blog, ESA, URL: http://blogs.esa.int/gaia/tag/ccd/
92) "Gaia video processing unit test model delivered," ESA, Feb. 13, 2009, URL: http://sci.esa.int/gaia/44228-gaia-video-processing-unit-test-model-delivered/
93) http://www.astrium.eads.net/en/equipment/gdpu-%E2%80%93-general-data-processing-unit.html
94) http://www.esa.int/Our_Activities/Operations/Gaia/%28print%29
95) David Milligan, Andreas Rudolph, Gary Whitehead, Tiago Loureiro, "Gaia Ground Segment Development and Operations Concept," AIAA SpaceOps 2010 Conference, Huntsville, AL, USA, April 25-30, 2010, Vol. 3, ISBN: 978-1-63266-002-2, URL: http://arc.aiaa.org/doi/pdf/10.2514/6.2010-2162
96) http://blogs.esa.int/gaia/
97) Rocio Guerra, "ESAC and the Gaia Catalogue," 2009, URL: http://www.ieec.cat/mediterrania2009/sites/default/files/proceedings/poster_Guerra.pdf
98) "Data Processing," ESA, URL: http://www.cosmos.esa.int/web/gaia/data-processing
99) Anthony G. A. Brown, "Gaia data processing," ESA, Sept. 23, 2013, URL: http://blogs.esa.int/gaia/2013/09/23/gaia-data-processing/
100) Veronique Valette, Kader Amsif, "CNES Gaia Data Processing Centre, a complex operation plan," Proceedings of SpaceOps 2012, The 12th International Conference on Space Operations, Stockholm, Sweden, June 11-15, 2012, URL: http://www.spaceops2012.org/proceedings/documents/id1291264-Paper-001.pdf
101) "List of Institutes involved in DPAC," ESA, URL: http://www.cosmos.esa.int/web/gaia/dpac/institutes
102) Veronique Valette, Benoit Frezouls, "Processing a billiom of star, an organizational challenge," SpaceOps 2014, 13th International Conference on Space Operations, Pasadena, CA, USA, May 5-9, 2014, paper: AIAA 2014-1701, URL: http://arc.aiaa.org/doi/pdf/10.2514/6.2014-1701
103) Christophe Arviset, Javier Durán, Juan González, Raúl Gutiérrez, José Hernández, Uwe Lammers, Bruno Merin, Sara Nieto, William OMullane, Jesús Salgado, Juan Carlos Segovia, "Big Data, Big Challenges and new Paradigm for the Gaia Archive," Proceedings of the 2016 Conference on Big Data from Space - BiDS'16, Santa Cruz de Tenerife, Spain, March 15-17, 2016, pp: 9-12, URL: http://publications.jrc.ec.europa.eu/repository/bitstream/JRC100655/LBNA27775ENN.pdf
The information compiled and edited in this article was provided by Herbert J. Kramer from his documentation of: "Observation of the Earth and Its Environment: Survey of Missions and Sensors" (Springer Verlag) as well as many other sources after the publication of the 4th edition in 2002. - Comments and corrections to this article are always welcome for further updates (eoportal@symbios.space).
Spacecraft Launch Mission Status Payload Module Ground Segment Big Data Archive References Back to top