XMM-Newton (X-ray Multi-Mirror Mission-Newton)
Non-EO
ESA
Quick facts
Overview
Mission type | Non-EO |
Agency | ESA |
Launch date | 10 Dec 1999 |
XMM-Newton (X-ray Multi-Mirror Mission-Newton) ObservatorySpacecraft Launch Mission Status Sensor Complement Ground Segment References
XMM-Newton has been one of the most successful astronomy missions launched by ESA (European Space Agency). It exploits innovative use of replication technology for the X-ray reflecting telescopes that has resulted in an unprecedented combination of effective area and resolution. Three telescopes are equipped with imaging cameras and spectrometers that operate simultaneously, together with a co-aligned optical telescope. The key features of the payload are described, and the in-orbit performance and scientific achievements are summarized. 1) 2) 3) 4)
Background: An X-ray astronomy mission for the European Space Agency Horizon 2000 program was studied from the early 1980’s, culminating in a mission presentation at an ESA workshop held in Lyngby, Denmark in June 1985. In the papers presented at this conference, the mission design contained 12 low-energy and 7 high-energy telescopes with a collecting area of 13000 cm2 and 10000 cm2 at 2 and 6 keV respectively. The scientific goal was to maximize the collecting area for spectroscopy, complementing the imaging science of the NASA AXAF program. When the report of the telescope working group was delivered in 1987, the consideration of practical constraints had reduced the number of telescopes to a more modest total of 7.
The mission was approved into implementation phase in 1994, and an improved observing efficiency achieved with a highly eccentric orbit allowed the number of telescopes to be reduced. The development of suitable mirrors involved parallel studies of solid nickel, nickel sandwich and carbon fiber technologies up to (as late as) early-1995. Soon afterwards the nickel electroforming replication technology was adopted, following the delivery of two successful mirror module demonstration models. The XMM flight model mirror modules were delivered in December 1998.
Mission objectives: Since Earth's atmosphere blocks out all X-rays, only a telescope in space can detect and study celestial X-ray sources. The XMM-Newton mission is helping scientists solve a number of cosmic mysteries, ranging from the enigmatic black holes to the origins of the Universe itself. Observing time on XMM-Newton is made available to the scientific community who apply to the regular announcements of opportunity on a competitive basis.
Mission name: The mission was initially known as XMM after its X-ray Multi-Mirror design, and was formally called the High Throughput X-ray Spectroscopy Mission because of its great capacity to detect X-rays. The name was later modified to XMM-Newton in honor of Sir Isaac Newton. — Announcing the new name on 9 February 2000, ESA's former Director of Science Prof. Roger Bonnet explained: "We have chosen this name because Sir Isaac Newton was the man who invented spectroscopy and XMM is a spectroscopy mission. The name of Newton is associated with the falling apple, which is the symbol of gravity and with XMM I hope that we will find a large number of black hole candidates which are of course associated with the theory of gravity. There was no better choice than XMM-Newton for the name of this mission".
A key aspect of the design was the simultaneous operation of 6 co-aligned instruments, the three EPIC (European Photon Imaging Camera) imaging X-ray cameras, the two RGS (Reflection Grating Spectrometer) grating X-ray spectrometers and the OM (Optical Monitor).
The XMM-Newton observatory provides unrivalled capabilities for detecting low surface brightness emission features from extended and diffuse galactic and extragalactic sources, by virtue of the large field of view of the X-ray telescopes and the high throughput yielded by the heavily nested telescope mirrors. In order to exploit the excellent EPIC data from extended objects, the EPIC background, now known to be higher than estimated pre-launch, needs to be understood thoroughly.
NASA cooperation: Besides having funded elements of the XMM-Newton instrument package, NASA also provides the NASA Guest Observer Facility (GOF) at NASA/GSFC (Goddard Space Flight Center). The GOF provides a clearing house for project-generated technical information and analysis software as well as budget support for U.S. astronomers who apply for XMM-Newton observation time. 5)
Some Science Highlights as of 2015
In orbit for more than 15 years, XMM-Newton has provided many insights into the workings of the universe, near and far. Here are a few examples (Ref. 5).
• Determined that Milky Way's Black Hole is believed to have woken up violently about 400 year ago and then turned off again about 100 years later.
• Identified the potential signatures of solar axioms, dark matter particle candidates.
• Measured the spin rate of a supermassive black hole for the first time in collaboration with NuSTAR.
• Acquired the first large-scale map of the dark matter and baryon distributions in the universe.
• Detected for the first time a switching X-ray emission when monitoring a highly variable pulsar - reopened the debate about the physical mechanisms powering the emission from pulsars.
• Discovered that the Orion Nebula contains a huge cloud of extremely hot gas, or plasma, heated to millions of degrees.
• Constructed the largest catalog of cosmic X-ray emitting objects.
• Showed that fierce winds from a supermassive black hole blow outward in all direction in collaboration with NuSTAR.
• Discovered 2XMM J083026+524133, the most massive cluster of galaxies seen in the distant Universe up to that time.
• Discovered the first definite detection of charge exchanged induced by X-ray emission on Mars.
• Acquired images of gamma-ray burst GRB 031203 that revealed the first detection of a time-dependent dust-scattered X-ray halo around a gamma-ray burst.
• Analyzed spectra of a distant active galaxy, 1H0707-495, which revealed two bright features of iron emission (the iron L and K lines) in the reflected X-rays that had never been seen together in an active galaxy.

Spacecraft
The XMM-Newton Observatory is a cornerstone mission of the European Space Agency's Horizon 2000 program, and is the largest scientific satellite it has launched to date. XMM-Newton is a three-axis stabilized spacecraft with a pointing accuracy of one arcsec. The satellite, which had a launch mass of 3800 kg, is made up of: a service module bearing the three X-ray mirror modules, propulsion and electrical systems, a long telescope tube and the focal plane assembly carrying the science instruments. The total length of XMM-Newton is 10 m, and its solar arrays give the satellite a 16 m span. The satellite was built under contract to ESA by the prime contractor Astrium, formerly Dornier Satellitensysteme (Friedrichshafen, Germany - part of DaimlerChrysler Aerospace), led an industrial consortium involving 46 companies from 14 European countries and one in the United States. Media Lario, Como, Italy, developed the X-ray mirror modules. - Although the nominal mission was for two years, XMM-Newton has been designed and built to perform well beyond that period. Mission operations have been extended until end-2018, with a mid-term review in 2016. 6) 7) 8)

The XMM spacecraft has a conventional structure and thermal design. Due to the long focal length of the telescopes (7.5 m), the mirrors are far removed from the instruments. On the ground and during the launch, the structure has to maintain the integrity of the whole spacecraft. The thermal control does not make use of onboard software. In orbit, the functions of the structure and the thermal control are mixed. Their global common requirement is to relate and align the set of mirrors at one end of the spacecraft with the set of instruments at the other.
TT (Telescope Tube): The TT maintains the relative position between the FPA (Focal Plane Array) and the MSP (Mirror Support Platform). Due to its length of 6.80 m, the Telescope Tube is physically composed of two halves: the upper and lower tubes. The upper tube includes two reversible VOD (Venting and Outgassing Doors), and supports the OGB (Outgassing Baffle).
TCS (Thermal Control Subsystem): The XMM satellite relies on a combination of passive and active means of thermal control. The passive thermal control is mainly achieved by using classical highly-insulating multi-layer blankets. Typically, blankets are made internally of 20 double-sided aluminized layers separated by Dacron nets. The external layer of all blankets is made of carbon-loaded Kapton, which gives the satellite its characteristic black appearance. This kind of Kapton has been chosen because of its electrical conductivity, which avoids electrostatic-discharge problems. In addition, the thermo-optical properties of the black finish will not change during the satellite's ten-year lifetime, helping again to maintain temperature stability. 9)
The insulation performance that has been achieved by the XMM blankets is exceptionally good, especially for the large, undisturbed blankets that insulate the telescope tube. Together with the black lining of its internal surface, they keep the temperature gradient across the tube diameter small and stable. In fact, the measured temperature difference across the tube was only 3°C. The telescope tube is not equipped with heaters and its temperature control is purely passive.

Focal-plane assembly compartment: The FPA compartment, located on top of the telescope tube and which contains the payload cameras, is controlled during operations in a totally passive way. This is made possible by the power dissipated by the instruments, which remains fairly constant during the mission observation periods. Consequently, the compartment's heat losses are trimmed such that the dissipated power (about 150 W) can keep the temperatures at the required level. Whenever an instrument chain is switched off, an appropriate ‘substitution’ heater line is switched on in order to replace the missing dissipated power and keep the heat power balance constant. In nonoperative and emergency modes, mechanical thermostats will switch these heaters if the temperature falls close to the non-operation temperature limits of the equipment.

SVM (Service Module): The SVM, accommodated at the other end of the telescope tube, is also fully blanketed with the exception of panel radiators. On the Sun-side they are covered by mirror solar reflectors, while those on the anti-sun side of the satellite are painted white. Where passive measures are not sufficient to meet the temperature requirements, heaters controlled by thermostats are implemented. No on-board software is used to activate and control heaters. Ground control can configure the heater lines to be powered, while mechanical thermostats perform the actual heater switching. In a typical mission observation phase, about 330 W are dissipated by the equipment and 80 W are provided by the heaters to maintain an internal average temperature of 15°C.
The configuration of the spacecraft is shown in an exploded view in Figure 5. It comprises 4 main sections (Ref. 1):

FPA (Focal Plane Assembly): The FPA, consisting of a platform carrying the focal-plane instruments: two RGS readout cameras, an EPIC PN and two EPIC MOS imaging detectors, and electronics boxes. The EPIC and RGS instruments are fitted with radiators, to passively cool the CCDs (Charge Coupled Devices).
MPS (Mirror Support Platform): The MSP, carrying the three mirror assemblies, the OM and the two star-trackers.
SVM (Service Module): The SVM,carrying the spacecraft subsystems, the two solar-array wings, the TSS (Telescope Sun Shield) and the two S-band antennae. The functions provided by the Service Module are:
- the primary and secondary structures to interface with the launcher adapter, to support the subsystem units, and to interface with and support the MSP (Mirror Support Platform) and the Telescope Tube
- the thermal control to maintain the SVM units and equipment within specified temperature limits, and to provide a very strictly controlled thermal environment for the mirror assemblies via the MZCU (Mirror Thermal Control Unit)
- the AOCS (Attitude and Orbit Control System) for precise pointing/slewing in all operational modes, and for the performance of orbit acquisition/maintenance via the RCS (Reaction Control System) propulsion system
- the OBDH (On-Board Data Handling) for the decoding of ground telecommands, the distribution of ground or on-board commands, the sampling and formatting of telemetry data, and central on-board time distribution
- the RFS (Radio Frequency System), operating in S-band, ensuring communications (uplinking of telecommands, downlinking of telemetry) with the ground stations and providing a ranging mode for orbit determination
- the EPS (Electrical Power Subsystem) for the generation and distribution of regulated power to all equipment via a 28 V main bus.

For reasons largely related to the mission design lifetime XMM-Newton has no onboard data storage capacity, so all data are immediately downloaded to the ground in real time, as facilitated by the ground station visibility. Contact for continuous real-time interaction with the spacecraft over almost the entire orbit is provided by the ESA ground stations at Perth and Kourou (with Villafranca and Santiago as back-up).
Mission operations are managed through the ESA/ESOC (European Spacecraft Operations Center) in Darmstadt, Germany. The observations are managed and archived at the ESAC (European Space Astronomy Center), formerly known as VILSPA) at Villafranca, Spain. The science data are processed at the XMM-Newton Survey Science Center at the University of Leicester, England.
Telescope Tube: The XMM observatory has, at its heart, three large X-ray telescopes, which will provide a large collecting area (1430 cm2 each at 1.5 keV, and 610 cm2 each at 8.0 keV) with a spatial resolution of around 14-15 arcsec. 10) 11)
The long carbon fiber Telescope Tube (not shown in Figure 5), maintaining the FPA and the MSP separation. The upper half includes reversible venting and out-gassing doors and baffles. The three telescopes each consist of the following elements, as shown in Figure 7:

- Mirror Assembly Door, which closed and protected the X-ray optics and the telescope interior against contamination until operations began.
- Entrance Baffle, which provides the visible stray light suppression.
- XRB (X-ray Baffle) which blocks X-rays from outside the nominal field of view, which would otherwise reflect once on the hyperboloid section of the mirrors and would therefore cause stray light.
- MM (Mirror Module) the X-ray optics themselves (Figure 8). The XMM mirrors were developed and manufactured by ESA and Media Lario (Bosisio Parini, Italy) with the support of: APCO (Vevey, Switzerland) for the manufacture of the structural parts and the containers; BCV-Progretti (Milan, Italy) for the optical and structural analysis; Kayser Threde (Munich, Germany) for the mechanical design and the analysis. The optical and X-ray stray-light work was conducted by ESA and Dornier (Friedrichshafen, Germany).
- Electron Deflector (producing a toroidal magnetic field for diverting "soft" electrons), right behind the mirrors in the shadow of the MM spider.
- RGA (Reflection Grating Assembly), with a mass of 60 kg, only present on the backside of two out of three MMs. It deflects roughly half of the X-ray light to a strip of CCD detectors (RGS), offset from the focal plane.
- Exit Baffle, providing a thermal environment for the gratings.
A Mirror Module is a Wolter 1 type grazing incidence telescope with a focal length of 7.5 m and with a resolution of ~15 arcsec (on-axis Half Energy Width). Each consists of 58 nested mirror shells bonded at one end on a spider. Design details are summarized in Table 1.
Focal length | 7500 mm |
Resolution (0.1-12 keV) HEW (Half Energy Width) | 15 arcsec (HEW), < 8 arcsec (FWHM) |
Effective area (1 keV) | 1500 cm2 |
Mirror diameter and thickness | Outermost:700 mm / 1.07 mm, Innermost: 306 mm / 0.47 mm |
Mirror length | 600 mm |
Packing distance | 1-5 mm |
Number of mirrors | 58 |
Reflective surface | Gold (250 nm layer) |
Mirror Module mass | 420 kg |
The X-ray mirrors are thin monolithic gold-coated nickel shells. The mirror shell manufacturing is based on a replication process, which transfers a gold layer deposited on the highly polished master mandrel to the electrolytic nickel shell, which is electroformed on the gold layer. The mandrels are made out of initially double conical aluminum blocks coated with Kanigen nickel and then lapped to the exact shape and finally super-polished to a surface roughness better than 4 Å (0.4 nm). The process is represented graphically in Figure 9.
In order to allow rapid testing of the individual mirror shells and integrated mirror modules a special vertical test facility (using a UV beam) was developed at the Centre Spatial de Liège in Belgium. The X-ray testing of the integrated XMM-Newton mirror modules was performed at the Panter facility of the MPE (Max-Planck Institut für Extraterrestrische Physik) Garching, Germany.




EPS (Electrical Power Subsystem): The principal mission and spacecraft characteristics influencing the design of XMM’s EPS were: 12)
- Power requirements: 1600 W in the sun, and 600 W in the eclipse phase of the orbit.
- Spacecraft geometry: Two separate PDUs (Power Distribution Units) to reduce harness mass, and to simplify system testing and AIV (Assembly, Integration and Verification), the Service and Focal-Plane Assembly Modules have dedicated the PDUs.
- Mirror stability: Leading to a dedicated MTCU (Mirror Thermal Control Unit).
The resulting EPS design is comprised of the following elements:
- A fixed two-wing deployable solar array for power generation.
- Two nickel-cadmium batteries.
- Two independent PDUs: one for the Focal-Plane Assembly (FPA PDU) and the other for the Service Module (SVM-PDU).
- A MTCU, dedicated to the thermal control of the mirror platform, Mirror Modules and Reflection Grating Assemblies.
- A PRU (Pyrotechnic Release Unit) for automatic activation of the release mechanisms for the solar arrays, and for the initial transmitter and AOCS activation.
The solar array has two wings, each with three rigid 1.94 m x 1.81 m panels, giving a total area of 21 m2 and a mass of 81.4 kg. The two wings are body-fixed and have a Sun incidence angle variation around normal of up to 28º. At end-of-life (EOL = 10 years), in the worst case, including one failed section, the solar array is required to provide 1600 W at 30 V at the interface connectors.
Battery: XMM has two identical 24 Ah nickel-cadmium batteries, each with 32 cells. Each 573 x 188 x 222 mm3 battery weighs 42 kg. To allow for cell short-circuits, the nominal energy budget is calculated with 31, rather than the full 32 cells. To allow for high peak power demands, a battery voltage higher than the bus voltage has been chosen, and battery reconditioning will be performed before each eclipse season.
MRU (Main Regulator Unit): The MRU provides a 28 V regulated main bus voltage, with protection to ensure uninterrupted operation even in the event of a single-point failure. During sunlit periods, the MRU provides power via S3Rs (Sequential Switching Shunt Regulators), as well as managing battery charging. In eclipse mode, the MRU controls the discharging of the two batteries to ensure correct current sharing. To reduce the power demand on the batteries and ensure that all non-essential loads are switched off during eclipse, an eclipse signal ECL is generated by the MRU and sent to the PDUs and MTCU. - The MRU provides 2 x 2 power lines for the SVM-PDU and the FPA-PDU, and 1 x 2 switched lines for the MTCU. For ground testing, it provides interfaces with solar-array and battery simulators.
OBDH (On-Board Data-Handling): OBDH is implemented in three internally redundant physical units: the CDMU (Central Data Management Unit) and two RTUs (Remote Terminal Units). The CDMU and one RTU are located on the Service Module of the spacecraft. The second RTU is installed on the FPA (Focal Plane Assembly). In addition to the RTUs and the CDMU, the OBDH includes six DBUs (Data Bus Units), which provide the scientific instruments with a digital interface to the data-handling services. 13)
The data processing in the CDMU is performed by the CTU (Central Terminal Unit) based on a MIL-STD-1750 microprocessor with 256 kwords of RAM. The packet handling functions, i.e. telemetry frame generation and telecommand frame decoding, are implemented in standard ASICs developed under ESA contracts, VCAs (Virtual Channel Assemblers), VCMs (Virtual Channel Mutiplexers) and PFDs (Packet Telecommand Decoders).
The users and all of the OBDH units are interconnected by the ESA OBDH bus, comprising a redundant set of interrogation bus and mono-directional response bus. The OBDH bus can transfer, depending on the command rate, approximately 200 kbit/s of telemetry data, which is roughly three times the XMM downlink data rate of 69.4 kbit/s (source packet level).
Packet protocol: Packet Terminals are connected to the OBDH via dedicated DBUs (Data Bus Units). The interface to the DBU, and thus the OBDH bus, is realized by an ASIC RBI (Remote Bus Interface). In order to have a common interface to all Packet Terminals, this specific RBI was imposed on all instruments and the AOCS (Attitude and Orbit Control Subsystem).
The RBI provides the CDMU with DMA (Direct Memory Access) to the processor memory of the Packet Terminals. In addition, the RBI accommodates registers for communication between the Packet Terminal and the OBDH and a register holding a copy of the onboard time.

RF subsystem: The RF subsystem is composed of three main blocks which are two Low Gain Antennas (LAG1 (+Z) and LAG2 (-Z)), two transponders and a Radio Frequency Distribution Network (RFDN) with two switches (SW-A and SW-T) to connect the transponders either to the LGA1 or LGA2. An S-Band transponder comprises three main modules: a diplexer, a receiver and a transmitter. The receiver assures the reception of signal in the range of 2025 to 2120 MHz and the phase demodulation of the telecommand signal and the ranging tones. The transmitter performs the modulation of the telemetry video signal and the ranging tones, as well as the power amplification of the output signal. The diplexer allows operating simultaneously the receiver and the transmitter with just a single RF connection (Ref. 153).
After the recovery from an antenna switch problem in 2008 it was agreed not to operate the faulty antenna switch (SW-A) of the RFDN anymore. A new strategy was implemented by switching between receivers and the corresponding transmitters. Apart from the SW-A the performance of the RF subsystem is very good. No degradation of any sub assembly was seen so far. The transmitter Latching Current Limiters (LCLs) that switch the transmitters on and off have been qualified for 50000 switchings. So far <900 switches for each transmitter have
been performed without any problems. The transmitter output level has not decreased significantly in comparison to the beginning of the mission.
RCS (Reaction Control Subsystem): XMM-Newton is equipped with a monopropellant RCS, with helium as pressurant and hydrazine as propellant. The propellant storage system consists of four tanks, three Auxiliary Tanks, which feed into the Main Tank, which in turn feeds the thrusters. The tanks of RCS are to load a total of 520 kg of hydrazine. The RCS consists of a 4 tank configuration, consisting of three Auxiliary Tanks with partial PMD (Propellant Management Device) feeding into one Main Tank with full PMD.
Autonomy: XMM is required to survive three days of ground-station outage. The autonomy on XMM is decentralized, meaning that all subsystems should manage their own survival and supply essential services to allow other subsystems to survive. Thus the central spacecraft autonomy function, often implemented in the data-handling subsystem, was not required for XMM. Recognizing this has allowed a substantial reduction in design and test expenditure by limiting the OBDH autonomy to protection against OBDH internal failures.

Launch
XMM was launched on December 10, 1999, via the first commercial Ariane-5 launch from Kourou, French-Guyana. It was the largest scientific European spacecraft to date; built and launched within the budget and schedule defined at approval. The overall mission cost was 689 MEuro (1999 economic conditions).
Orbit: HEO (Highly-elliptical Earth Orbit ). After launch, the spacecraft was placed into a 48 hour elliptical orbit around the Earth, with an inclination of 40º, a southern apogee at an altitude of 114 000 km, and a perigee altitude of 7000 km. The orbital parameters evolve as the mission progresses. As an example, the perigee altitude has varied between 6000 km and 22 000 km, while the apogee altitude has varied between 99 000 km and 115 000 km. However, the orbital period is always kept at 48 hours.
XMM-Newton's operational orbit was chosen for two reasons: First, the XMM-Newton instruments need to work outside the radiation belts surrounding the Earth. Second, a highly eccentric orbit offers the longest possible observation periods - less interrupted by the frequent passages in the Earth's shadow that occur in LEO (Low Earth Orbit). In addition, the orbital period of XMM-Newton is exactly two times the Earth rotation period to maintain optimal contact between XMM-Newton and the ground stations tracking the satellite. This allows XMM-Newton data to be received in real-time and for it to be fed to the Mission Control Centers.

Mission Status
• July 4, 2022: ESA’s XMM-Newton has X-rayed this beautiful cosmic creature, known as the Manatee Nebula, pinning down the location of unusual particle acceleration in its ‘head’. 16)
- The Manatee Nebula, or W50, is thought to be a large supernova remnant created when a giant star exploded around 30,000 years ago, flinging its shells of gases out across the sky. It is one of the largest such features known, spanning the equivalent size of four full Moons.
- Unusually for a supernova remnant, a black hole remains in its core. This central ‘microquasar’, known as SS 433, emits powerful jets of particles travelling at speeds close to a quarter the speed of light that punch through the gassy shells, creating the double-lobed shape.
- SS 433 is identified by the red dot in the middle of the image. The X-ray data acquired by XMM-Newton are represented in yellow (soft X-rays), magenta (medium energy X-rays) and cyan (hard X-ray emission), while red is radio and green optical wavelengths imaged by the Very Large Array and the Skinakas Observatory in Greece, respectively. NASA NuSTAR and Chandra data were also used for the study - not shown in this image.
- The nebula attracted attention in 2018 when the High-Altitude Water Cherenkov Observatory (HAWC), which is sensitive to very high energy gamma-ray photons, revealed the presence of highly energetic particles (hundreds of tera electron volts), but could not pinpoint from where within the Manatee the particles were originating.
- XMM-Newton was crucial in homing in on the region of particle acceleration in the X-ray jet blasting from the Manatee’s head, which begins about 100 light years away from the microquasar (represented by the magenta and cyan colours towards the left side SS 433) and extends to approximately 300 light years (coinciding with the radio ‘ear’ where the shock terminates).
- Samar Safi-Harb of the University of Manitoba, Canada, who led the study, says “thanks to the new XMM-Newton data, supplemented with NuSTAR and Chandra data, we believe the particles are getting accelerated to very high energies in the head of the Manatee through an unusually energetic particle acceleration process. The black hole outflow likely made its way there and has been re-energized to high-energy radiation at that location, perhaps due to shock waves in the expanding gas clouds and enhanced magnetic fields.” 17)
- The nebula acts as a nearby laboratory for exploring a wide range of astrophysical phenomena associated with the outflows of many galactic and extragalactic sources and will be subject to further investigation. Furthermore, follow-up studies by ESA’s future Athena X-ray observatory will provide even more sensitive details about the inner workings of this curious cosmic Manatee.
![Figure 16: Multi-wavelength image of the W50 nebula. Red: Radio (Dubner et al. 1998), Green: Optical (Boumis et al. 2007), Yellow: Soft X-rays (0.5–1 keV), Magenta: Medium energy X-rays (1–2 keV), Cyan: Hard X-ray emission (2–12 keV). The eastern lobe X-ray image highlights the XMM-Newton data presented in this work (with the brightening at the edge of the field of view cropped here to highlight the source emission). The western lobe X-ray image shows only partial Chandra coverage of part of the nebula (Moldowan et al. 2005); additional X-ray observations of this and other regions are currently being carried out and will be presented in future work [image credit: S. Safi-Harb, et al. (2022)]](https://www.eoportal.org/ftp/satellite-missions/x/XMM-Newton_040722/XMM-Newton_Auto7A.jpeg)
• October 25, 2021: Using ESA’s XMM-Newton and NASA’s Chandra X-ray space telescopes, astronomers have made an important step in the quest to find a planet outside of the Milky Way. 18) 19)
- Spotting a planet in another galaxy is hard, and even though astronomers know that they should exist, no planetary systems outside of the Milky Way have been confirmed so far. Because the light from another galaxy is packed into a tiny area on the sky, it is very difficult for telescopes to distinguish one star from another, let alone a planet orbiting around them. And the usual techniques to find exoplanets in our galaxy don’t work as well for planets outside of it.
- This is different when studying X-rays, instead of visible light, in a galaxy. Because there are less objects that shine bright in X-ray light, an X-ray telescope like ESA’s XMM can more easily distinguish between objects when observing a galaxy. Those objects are therefore easier to identify and study, and it might be possible to find a planet around them.
- Some of the brightest objects that can be studied in external galaxies are the so-called X-ray binaries. They consist of a very compact object – a neutron star or black hole – that is eating material from a companion, or ‘donor’, star orbiting around it. The infalling material is accelerated by the intense gravitational field of the neutron star or black hole and heated to millions of degrees, producing a lot of bright X-rays. Astronomers expect that theoretically, planets passing in front of (transiting) such a source would block these X-rays, causing a dip in the observed X-ray light curve.


- “X-ray binaries may be ideal places to search for planets, because, although they are a million times brighter than our Sun, the X-rays come from a very small region. In fact, the source that we studied is smaller than Jupiter, so a transiting planet could completely block the light from the X-ray binary,” explains Rosanne Di Stefano from the Center for Astrophysics, Harvard & Smithsonian in the United States, and first author of a new study published in Nature Astronomy today.
- Rosanne and colleagues searched in Chandra and XMM-Newton data of three galaxies for such X-ray transits, dips in the light that could be explained by planets. And they found a very special signal in the Whirlpool Galaxy (M51) that they decided to study in more detail. The dip occurred in X-ray binary M51-ULS-1 and completely blocked the signal for a few hours, before it came back again.
- Now the game of carefully crossing off possible explanations began, before the researchers could even consider the option of an extragalactic planet. “We first had to make sure that the signal was not caused by anything else,” says Rosanne, whose team argues against a number of possibilities in their new publication. “We did this by an in-depth analysis of the X-ray dip in the Chandra data, analyzing other dips and signals in the XMM data, and also modelling dips caused by other possible events, including a planet.”


- Could the X-ray dip be caused by small stars like a brown or red dwarf? No, they argue, the system is too young for that, and the transiting object too large.
- Could it be a cloud of gas and dust? Not likely, the team says, because the dip indicates a transiting object with a well-defined surface, which would not be the same for a passing cloud. Even if the planet had an atmosphere, it would still have a more well-defined surface than a cloud.
- Could the dip be explained by variations in brightness of the source itself? The paper authors are confident that this is not the case, because although the light from the source completely disappeared for a few hours before it came back, the temperature and light colors stayed the same.
- Lastly, the team also compared the dip to another blockage of the light caused by the ‘donor’ star passing in front of the compact star. This was partly observed by XMM-Newton and caused a much longer black-out, which was different from the dip caused by a possible planet.
- “We did computer simulations to see whether the dip has the characteristics of a planet transiting, and we find that it fits perfectly. We are pretty confident that this is not anything else and that we have found our first planet candidate outside of the Milky Way,” adds Rosanne.
- The team also speculates about the characteristics of the planet based on their observations: it would be the size of Saturn, orbiting the binary star system from tens of times the Earth-Sun distance. It would make one full orbit roughly every 70 years, and be bombarded with extreme amounts of radiation, making it inhabitable by life as we know it on Earth.
- This long orbit of the planet candidate is also a limitation for the study, because the event can’t be repeated any time soon. That’s why the team remains careful to say that they found a possible planet candidate, for which the broader community might find other explanations, although they have not been found after careful research by the team. “We can only say with confidence that it doesn’t fit any of our other explanations,” Rosanne clarifies.
- Still, this is an exciting step forward in the quest to find a planet outside of the Milky Way. This is the first planet candidate that would orbit a known host system, as compared to candidates found with gravitational lenses. This would also be the first time that a planet is found orbiting an X-ray binary. The existence of those planets is consistent with the fact that planets are found around pulsars (rapidly rotating neutron stars), and some of these pulsars have been part of an X-ray binary in the past.
- “The first confirmed planet outside of our Solar System was found around a pulsar, an object typically observed in X-rays. I am excited that X-rays now also play important step in the search for planets beyond the border of our galaxy,” says Norbert Schartel, XMM-Newton Project Scientist for ESA. 20)
- “Now that we have this new method for finding possible planet candidates in other galaxies, our hope is that by looking at all the available X-ray data in the archives, we find many more of those. In the future we might even be able to confirm their existence,” says Rosanne.
- ESA’s quest to finding exoplanets continues with Cheops, Gaia, and future missions Webb, Plato and Ariel. 21)
• July 28, 2021: For the first time, astronomers have seen light coming from behind a black hole. 22)
- Using ESA’s XMM-Newton and NASA’s NuSTAR space telescopes, an international team of scientists led by Dan Wilkins of Stanford University in the USA observed extremely bright flares of X-ray light coming from around a black hole.

- The astronomers did not expect to see anything from behind the black hole, since no light can escape from it. But because of the black hole’s extreme gravity warping the space around it, light echoes from behind the black hole were bent around the black hole, making them visible from XMM and NuSTAR’s point of view.
- The discovery began with the search to find out more about the mysterious ‘corona’ of the black hole, which is the source of the bright X-ray light. Astronomers think that the corona is a result of gas that falls continuously into the black hole, where it forms a spinning disk around it – like water flushing down a drain.
- This gas disk is heated up to millions of degrees and generates magnetic fields that get twisted into knots by the spinning black hole. When the magnetic field gets tied up, it eventually snaps, releasing the energy stored within it. This heats everything around it and produces the corona of high energy electrons that produce the X-ray light.
- The X-ray flare observed from I Zwicky 1 was so bright that some of the X-rays shone down onto the disk of gas falling into the black hole. The X-rays that reflected on the gas behind the black hole were bent around the black hole, and these smaller flashes arrived at the telescopes with a delay. These observations match Einstein’s predictions of how gravity bends light around black holes, as described in his theory of General Relativity.
- The echoes of X-rays from the disk have specific ‘colors’ of light and as the X-rays travel around the black hole, their colors change slightly. Because the X-ray echoes have different colors and are seen at different times, depending where on the disk they reflected from, they contain a lot of information about what is happening around a black hole. The astronomers want to use this technique to create a 3D map of the black hole surroundings.
- Another mystery to be solved in future studies is how the corona produces such bright X-ray flares. The mission to characterize and understand black hole coronas will continue with XMM-Newton and ESA’s future X-ray observatory, Athena (Advanced Telescope for High-ENergy Astrophysics).
- The team published their findings in Nature. 23)
• July 13, 2021: A puzzler about the gas giant’s intense northern and southern lights has been deciphered. 24)
![Figure 23: The purple hues in this image show X-ray emissions from Jupiter’s auroras, detected by NASA’s Chandra Space Telescope in 2007. They are overlaid on an image of Jupiter taken by NASA’s Hubble Space Telescope. Jupiter is the only gas giant planet where scientists have detected X-ray auroras [image credits: (X-ray) NASA/CXC/SwRI/R. Gladstone et al.; (Optical) NASA/ESA/Hubble Heritage (AURA/STScI)]](https://www.eoportal.org/ftp/satellite-missions/x/XMM-Newton_040722/XMM-Newton_Auto74.jpeg)
- Planetary astronomers combined measurements taken by NASA’s Juno spacecraft orbiting Jupiter, with data from ESA’s (the European Space Agency’s) Earth-orbiting XMM-Newton mission, to solve a 40-year-old mystery about the origins of Jupiter’s unusual X-ray auroras. For the first time, they have seen the entire mechanism at work: The electrically charged atoms, or ions, responsible for the X-rays are “surfing” electromagnetic waves in Jupiter’s magnetic field down into the gas giant’s atmosphere.
- A paper on the study was published on July 9 in the journal Science Advances. 25)
- Auroras have been detected on seven planets in our solar system. Some of these light shows are visible to the human eye; others generate wavelengths of light we can only see with specialized telescopes. Shorter wavelengths require more energy to produce. Jupiter has the most powerful auroras in the solar system and is the only one of the four giant planets with an aurora that has been found to emit X-rays.
- Planetary astronomers have been fascinated with Jupiter’s X-ray auroral emission since its discovery four decades ago because it was not immediately clear how the energy required to produce it is generated. They knew these surprising Jovian northern and southern lights are triggered by ions crashing into Jupiter’s atmosphere. But until now scientists had no idea how the ions responsible for the X-ray light show are able to get to the atmosphere in the first place.
- At Earth, auroras are usually visible only in a belt surrounding the magnetic poles, between 65 and 80 degrees latitude. Beyond 80 degrees, auroral emission disappears because the magnetic field lines leave Earth and connect to the magnetic field in the solar wind, which is the constant flux of electrically charged particles ejected by the Sun. These are called open field lines, and in the traditional picture, Jupiter’s and Saturn’s high-latitude polar regions are not expected to emit substantial auroras, either.
- However, Jupiter’s X-ray auroras are different. They exist poleward of the main auroral belt and pulsate, and those at the north pole often differ from those at the south pole. These are typical features of a closed magnetic field, where the magnetic field line exits the planet at one pole and reconnects with the planet at the other. All planets with magnetic fields have both open and closed field components.
- However, Jupiter’s X-ray auroras are different. They exist poleward of the main auroral belt and pulsate, and those at the north pole often differ from those at the south pole. These are typical features of a closed magnetic field, where the magnetic field line exits the planet at one pole and reconnects with the planet at the other. All planets with magnetic fields have both open and closed field components.
- Scientists studying the phenomena turned to computer simulations and found that the pulsating X-ray auroras could be linked to closed magnetic fields that are generated inside Jupiter and then stretch out millions of miles into space before turning back. But how to prove the model was viable?
- The study authors turned to data acquired by both Juno and XMM-Newton from July 16 to 17, 2017. During the two-day span, XMM-Newton observed Jupiter continuously for 26 hours and saw X-ray aurora pulsating every 27 minutes.
- At the same time, Juno had been traveling between 62 and 68 Jupiter radii (about 2.8 to 3 million miles, or 4.4 to 4.8 million kilometers) above the planet’s pre-dawn area. This was exactly the region that the team’s simulations suggested was important for triggering the pulsations, so they searched the Juno data for any magnetic processes that were occurring at the same rate.
- They found that fluctuations of Jupiter’s magnetic field caused the pulsating X-ray auroras. The outer boundary of the magnetic field is struck directly by the particles of the solar wind and compressed. These compressions heat ions that are trapped in Jupiter’s extensive magnetic field, which are millions of miles away from the planet’s atmosphere.
- “What we see in the Juno data is this beautiful chain of events. We see the compression happen, we see the EMIC wave triggered, we see the ions, and then we see a pulse of ions traveling along the field line,” said William Dunn of the Mullard Space Science Laboratory, University College London, and a co-author of the paper. “Then, a few minutes later, XMM sees a burst of X-rays.”
- Now that the missing piece of the process has been identified for the first time, it opens up a wealth of possibilities for where it could be studied next. For example, at Jupiter, the magnetic field is filled with sulfur and oxygen ions being emitted by the volcanoes on the moon Io. At Saturn, the moon Enceladus jets water into space, filling Saturn’s magnetic field with water group ions.
• July 9, 2021: The 40-year-old mystery of what causes Jupiter’s X-ray auroras has been solved. For the first time, astronomers have seen the entire mechanism at work – and it could be a process occurring in many other parts of the Universe too. 26)
- Planetary astronomers have studied Jupiter’s spectacular X-ray auroral emission for decades. The X-ray ‘colors’ of these auroras show that they are triggered by electrically charged particles called ions crashing into Jupiter’s atmosphere. But astronomers had no idea how the ions were able to get to the atmosphere in the first place.
- Now, for the first time, they have seen the ions ‘surfing’ electromagnetic waves in Jupiter’s magnetic field, down into the atmosphere.

- The vital clues came from a new analysis of data from ESA’s XMM-Newton telescope and NASA’s Juno spacecraft. Situated in Earth’s orbit, XMM-Newton makes remote observations of Jupiter at X-ray wavelengths. Juno on the other hand circles the giant planet itself, taking in-situ readings from inside Jupiter’s magnetic field. But the question was: what should the team look for?
- The clue came when Zhonghua Yao, Institute of Geology and Geophysics, Chinese Academy of Sciences, Beijing, and lead author of the new study, realized that something didn’t make sense about Jupiter’s X-ray auroras.
- On Earth, auroras are visible only in a belt surrounding the magnetic poles, between 65 and 80 degrees latitude. Beyond 80 degrees, auroral emission disappears because the magnetic field lines here leave Earth and connect to the magnetic field in the solar wind, which is the constant flux of electrically charged particles ejected by the Sun. These are called open field lines and in the traditional picture, Jupiter and Saturn’s high-latitude polar regions are not expected to emit substantial auroras.
- However, Jupiter’s X-ray auroras are inconsistent with this picture. They exist poleward of the main auroral belt, pulsate regularly, and can sometimes be different at the north pole from the south pole. These are typical features of a ‘closed’ magnetic field, where the magnetic field line exits the planet at one pole and reconnects with the planet at the other.
- Using computer simulations, Zhonghua and colleagues previously found that the pulsating X-ray auroras could be linked to closed magnetic fields that are generated inside Jupiter and then stretch out millions of kilometers into space before turning back. 27)
- On 16 and 17 July 2017, XMM-Newton observed Jupiter continuously for 26 hours and saw X-ray auroras pulsating every 27 minutes. Simultaneously, Juno had been travelling between 62 and 68 Jupiter radii above the planet’s pre-dawn areas. This was exactly the area that the team’s simulations suggested were important for triggering the pulsations. So, the team searched the Juno data for any magnetic processes that were occurring at the same rate.
- They found that the pulsating X-ray auroras are caused by fluctuations of Jupiter’s magnetic field. As the planet rotates, it drags around its magnetic field. The magnetic field is struck directly by the particles of the solar wind and compressed. These compressions heat particles that are trapped in Jupiter’s magnetic field. This triggers a phenomenon called electromagnetic ion cyclotron (EMIC) waves, in which the particles are directed along the field lines.
- The particles themselves are electrically charged atoms called ions. Guided by the field, the ions ‘surf’ the EMIC wave across millions of kilometers of space, eventually slamming into the planet’s atmosphere and triggering the X-ray aurora.
- “What we see in the Juno data is this beautiful chain of events. We see the compression happen, we see the EMIC wave triggered, we see the ions, and then we see a pulse of ions traveling along the field line. And then a few minutes later, XMM sees a burst of X-rays,” says William Dunn, Mullard Space Science Laboratory, University College London, who co-led the research.
- Now that the process responsible for Jupiter’s X-ray auroras has been identified for the first time, it opens up a wealth of possibilities for where it could be studied next. For example, at Jupiter, the magnetic field is filled with sulphur and oxygen ions that are spewed out by the volcanoes on the moon Io. At Saturn, the moon Enceladus jets water into space, filling Saturn’s magnetic field with water ions.
- “This is a fundamental process that’s applicable to Saturn, Uranus, Neptune and probably exoplanets as well,” says Zhonghua.
- It may be more widely applicable even than that because now that the process has been revealed, there is a striking similarity to the ion auroras that happen here on Earth. In the case of Earth, the ion responsible is a proton, which comes from a hydrogen atom, and the process is not energetic enough to create X-rays. Yet, the basic process is that same. So, Jupiter’s X-ray aurora is fundamentally an ion aurora, although at much higher energy than the proton aurora on Earth.
- “It could be that EMIC waves play an important role in transferring energy from one place to another across the cosmos,” says William.
- As for Jupiter itself, the study of its auroras will continue with ESA’s JUpiter ICy moons Explorer (JUICE). Set to arrive by 2029, Juice will study the planet’s atmosphere, magnetosphere, and the effect that Jupiter’s four largest moons have on the auroras.
• June 29, 2021: New observations made with ESA’s X-ray XMM Newton telescope have revealed an “orphan cloud” – an isolated cloud in a galaxy cluster that is the first discovery of its kind. 28)
- A lot goes on in a galaxy cluster. There can be anything from tens to thousands of galaxies bound together by gravity. The galaxies themselves have a range of different properties, but typically contain systems with stars and planets, along with the material in between the stars – the interstellar medium. In between the galaxies is more material – tenuous hot gas known as the intercluster medium. And sometimes in all the chaos, some of the interstellar medium can get ripped out of a galaxy and get stranded in an isolated region of the cluster, as this new study reveals.
Unexpected Discovery
- Abell 1367, also known as the Leo Cluster, is a young cluster that contains around 70 galaxies and is located around 300 million light-years from Earth. In 2017, a small warm gas cloud of unknown origin was discovered in A1367 by the Subaru telescope in Japan. A follow-up X-ray survey to study other aspects of A1367 unexpectedly discovered X-rays emanating from this cloud, revealing that the cloud is actually bigger than the Milky Way.

- The discovery of this orphan cloud was made by Chong Ge at the University of Alabama in Huntsville, and colleagues, and the study has been published in Monthly Notices of the Royal Astronomical Society. 29)
- Along with data from XMM-Newton and Subaru, Chong and colleagues also used the Multi Unit Spectroscopic Explorer (MUSE) on the Very Large Telescope (VLT) to observe the cluster in visible light.
- The orphan cloud is the blue umbrella-shaped part of the image. It has been color-coded to show the X-ray part of the cloud in blue, the warm gas in red, and the visible region in white shows some of the galaxies in the cluster. The part of the cloud that had been discovered in 2017 (in red) overlaps with the X-ray at the bottom of the cloud.
How the Cloud Became an Orphan
- It was previously thought that the distribution of material between galaxies is smooth, however more recent X-ray studies have revealed the presence of clumps in clusters. It was theorized that clumps of gas in the clusters were originally the gas that exists between stars in individual galaxies. The intercluster gas acts as a wind that is strong enough to pull the interstellar gas out of the galaxy as the galaxy is moving through the cluster. However, observations showing that intercluster clumps are originally stripped interstellar material have never been made until now. The observation of the warm gas in the clump provides the evidence to show that this orphan cloud originated within a galaxy. Interstellar material is much cooler than intercluster material, and the temperature of the orphan cloud matches that of interstellar gas. The researchers were also able to determine why the orphan cloud has survived for as long as it has. An isolated cloud would be expected to be ripped apart by instabilities caused by velocity and density differences. However, they found that a magnetic field in the cloud would be able to suppress these instabilities.
Searching for the Parent Galaxy
- It is likely that the parent galaxy of the orphan cloud is a massive one as the mass of the X-ray gas in the orphan is substantial. It is possible that the parent might one day be discovered with future observations by following some breadcrumbs. For example, there are traces of the warm gas that extend beyond the orphan cloud that could be used to identify the parent with more data. There are other unsolved mysteries regarding the cloud that could be deciphered with more observations, such as mysterious offset between the brightest X-rays and the brightest light from the warm gas.
- A closer inspection of this orphan will also further our understanding of the evolution of stripped interstellar medium at such a great distance from its parent galaxy and will provide a rare laboratory to study other things such as turbulence and heat conduction. This study paves the way for research on intercluster clumps, as future warm gas surveys can now be targeted to search for other orphan clouds.
• January 15, 2021: A new study, led by a theoretical physicist at the U.S. Department of Energy’s Lawrence Berkeley National Laboratory (Berkeley Lab), suggests that never-before-observed particles called axions may be the source of unexplained, high-energy X-ray emissions surrounding a group of neutron stars. 30)
- First theorized in the 1970s as part of a solution to a fundamental particle physics problem, axions are expected to be produced at the core of stars, and to convert into particles of light, called photons, in the presence of a magnetic field.
- Axions may also make up dark matter – the mysterious stuff that accounts for an estimated 85 percent of the total mass of the universe, yet we have so far only seen its gravitational effects on ordinary matter. Even if the X-ray excess turns out not to be axions or dark matter, it could still reveal new physics.
- A collection of neutron stars, known as the Magnificent 7, provided an excellent test bed for the possible presence of axions, as these stars possess powerful magnetic fields, are relatively nearby – within hundreds of light-years – and were only expected to produce low-energy X-rays and ultraviolet light.
- “They are known to be very ‘boring,’” and in this case it’s a good thing, said Benjamin Safdi, a Divisional Fellow in the Berkeley Lab Physics Division theory group who led a study, published Jan. 12 in the journal Physical Review Letters, detailing the axion explanation for the excess. Christopher Dessert, a Berkeley Lab Physics Division affiliate, contributed heavily to the study, which also had participation by researchers at UC Berkeley, the University of Michigan, Princeton University, and the University of Minnesota. 31)
- If the neutron stars were of a type known as pulsars, they would have an active surface giving off radiation at different wavelengths. This radiation would show up across the electromagnetic spectrum, Safdi noted, and could drown out this X-ray signature that the researchers had found, or would produce radio-frequency signals. But the Magnificent 7 are not pulsars, and no such radio signal was detected. Other common astrophysical explanations don’t seem to hold up to the observations either, Safdi said.
- If the X-ray excess detected around the Magnificent 7 is generated from an object or objects hiding out behind the neutron stars, that likely would have shown up in the datasets that researchers are using from two space satellites: the European Space Agency’s XMM-Newton and NASA’s Chandra X-ray telescopes.
- Safdi and collaborators say it’s still quite possible that a new, non-axion explanation arises to account for the observed X-ray excess, though they remain hopeful that such an explanation will lie outside of the Standard Model of particle physics, and that new ground- and space-based experiments will confirm the origin of the high-energy X-ray signal.
- “We are pretty confident this excess exists, and very confident there’s something new among this excess,” Safdi said. “If we were 100% sure that what we are seeing is a new particle, that would be huge. That would be revolutionary in physics.” Even if the discovery turns out not to be associated with a new particle or dark matter, he said, “It would tell us so much more about our universe, and there would be a lot to learn.”
- Raymond Co, a University of Minnesota postdoctoral researcher who collaborated in the study, said, “We’re not claiming that we’ve made the discovery of the axion yet, but we’re saying that the extra X-ray photons can be explained by axions. It is an exciting discovery of the excess in the X-ray photons, and it’s an exciting possibility that’s already consistent with our interpretation of axions.”
- If axions exist, they would be expected to behave much like neutrinos in a star, as both would have very slight masses and interact only very rarely and weakly with other matter. They could be produced in abundance in the interior of stars. Uncharged particles called neutrons move around within neutron stars, occasionally interacting by scattering off of one another and releasing a neutrino or possibly an axion. The neutrino-emitting process is the dominant way that neutron stars cool over time.
![Figure 27: An artistic rendering of the XMM-Newton (X-ray Multi-Mirror Mission) space telescope. A study of archival data from the XMM-Newton and the Chandra X-ray space telescopes found evidence of high levels of X-ray emission from the nearby Magnificent Seven neutron stars, which may arise from the hypothetical particles known as axions [image credits: D. Ducros, ESA/XMM-Newton, CC BY-SA 3.0 IGO)]](https://www.eoportal.org/ftp/satellite-missions/x/XMM-Newton_040722/XMM-Newton_Auto71.jpeg)
- Like neutrinos, the axions would be able to travel outside of the star. The incredibly strong magnetic field surrounding the Magnificent 7 stars – billions of times stronger than magnetic fields that can be produced on Earth – could cause exiting axions to convert into light.
- Neutron stars are incredibly exotic objects, and Safdi noted that a lot of modeling, data analysis, and theoretical work went into the latest study. Researchers have heavily used a bank of supercomputers known as the Lawrencium Cluster at Berkeley Lab in the latest work.
- Some of this work had been conducted at the University of Michigan, where Safdi previously worked. “Without the high-performance supercomputing work at Michigan and Berkeley, none of this would have been possible,” he said. “There is a lot of data processing and data analysis that went into this. You have to model the interior of a neutron star in order to predict how many axions should be produced inside of that star.”
- Safdi noted that as a next step in this research, white dwarf stars would be a prime place to search for axions because they also have very strong magnetic fields, and are expected to be “X-ray-free environments.”
- “This starts to be pretty compelling that this is something beyond the Standard Model if we see an X-ray excess there, too,” he said.
- Researchers could also enlist another X-ray space telescope, called NuStar, to help solve the X-ray excess mystery.
- Safdi said he is also excited about ground-based experiments such as CAST at CERN, which operates as a solar telescope to detect axions converted into X-rays by a strong magnet, and ALPS II in Germany, which would use a powerful magnetic field to cause axions to transform into particles of light on one side of a barrier as laser light strikes the other side of the barrier.
- Axions have received more attention as a succession of experiments has failed to turn up signs of the WIMP (weakly interacting massive particle), another promising dark matter candidate. And the axion picture is not so straightforward – it could actually be a family album.
- There could be hundreds of ALPs (Axion-Like Particles) that make up dark matter, and string theory – a candidate theory for describing the forces of the universe – holds open the possible existence of many types of ALPs.
- The study was supported by the U.S. Department of Energy Office of Science Early Career Research Program; Advanced Research Computing and the Leinweber Graduate Fellowship at the University of Michigan, Ann Arbor; the National Science Foundation; the Mainz Institute for Theoretical Physics (MITP) of the Cluster of Excellence PRISMA+; the Munich Institute for Astro- and Particle Physics (MIAPP) of the DFG Excellence Cluster Origins; and the CERN Theory department.
• January 11, 2021: A team of astronomers led by Lidia Oskinova of the University of Potsdam, Germany, used ESA’s XMM-Newton X-ray telescope to study the object that was originally discovered in 2019. Back then, astronomers already reported that the object has very high wind speeds and is too bright, and therefore too massive, to be an ordinary white dwarf. They suggested that the object is a new type of star that survived the merger of two white dwarfs. 32)
- Based on new information from XMM-Newton, Lidia and her team now suggest that what we see in the image is a new type of X-ray source powered by the merger of two white dwarfs. The remnant of the clash – the nebula – is also visible in this image, and is mostly made out of the element neon (shown in green). The star is very unstable and will likely collapse into a neutron star within 10,000 years. 33)

• November 12, 2020: This burst of color shows a fascinating discovery: a galaxy cluster acting as a cosmic furnace. The cluster is heating the material within to hundreds of millions of degrees Celsius – well over 25 times hotter than the core of the Sun. 34)
- The cluster, named HSC J023336-053022 (XLSSC 105), lies four billion light-years from Earth and was independently discovered by both ESA’s spaceborne XMM-Newton X-ray Observatory and NAOJ’s Subaru optical-infrared telescope in Hawaii, USA. XMM-Newton detected the cluster via the international XXL survey, which is exploring two large areas of space outside our galaxy.
- Galaxies are not distributed randomly throughout the Universe, and instead exist within groups and larger clusters. These aggregations can be mammoth and sometimes contain many thousands of individual galaxies in a single structure, all embedded in clumps of invisible dark matter. Different sub-groups of galaxies can also form within a single cluster, as shown here by the two blue-purple circles on either side of centre. These circles mark the locations of two sub-clusters within HSC J023336-053022 which are slowly moving towards and colliding with one another, ‘shock heating’ gas to intense temperatures in the process.
- The addition of radio observations makes this image special, as many studies of collisions within or between galaxy clusters have not captured this shock-heating process – which is represented visually in the region where green changes to red – in radio. This process releases immense amounts of energy and heats already scorching gas to temperatures tens of times hotter. Before shock heating, the gas sits at around 40 million degrees Celsius – already some 2.7 times hotter than the core of the Sun.
![Figure 29: To create this image, three different international teams of astronomers explored observations of the cluster across the electromagnetic spectrum, in order to isolate and pinpoint different aspects of this region of space. These aspects are shown here in different colors. Individual galaxies within the cluster show up in orange, and dark matter – which maps the location of the two sub-clusters – in blue (via optical observations from Subaru). Hot, dense gas shows up in green (X-ray from XMM-Newton), while hot, thin, high-pressure gas shows up in red (radio from the Green Bank Telescope in Virginia, USA). This gas is something known as the ‘intracluster medium’, which permeates galaxy clusters and fills the space between galaxies [image credit: Radio: GBT Green Bank Observatory/National Science Foundation (NSF); Optical: Subaru Telescope, National Astronomical Observatory of Japan/HSC-SSP collaboration; X-ray: European Space Agency (ESA)/XMM-Newton/XXL survey consortium]](https://www.eoportal.org/ftp/satellite-missions/x/XMM-Newton_040722/XMM-Newton_Auto6F.jpeg)
• June 17, 2020: An observation campaign led by ESA’s XMM-Newton space observatory reveals the youngest pulsar ever seen – the remnant of a once-massive star – that is also a ‘magnetar’, sporting a magnetic field some 70 quadrillion times stronger than that of Earth. 35)
- Pulsars are some of the most exotic objects in the Universe. They form as massive stars end their lives via powerful supernova explosions and leave extreme stellar remnants behind: hot, dense and highly magnetized. Sometimes pulsars also undergo periods of greatly enhanced activity, in which they throw off enormous amounts of energetic radiation on timescales from milliseconds to years.
- Smaller bursts often mark the onset of a more enhanced ‘outburst’, when X-ray emission can become a thousand times more intense. A multi-instrument campaign led by XMM-Newton has now captured such an outburst emanating from the youngest baby pulsar ever spotted: Swift J1818.0-1607, which was originally discovered by NASA’s Swift Observatory in March.
- And there is more. Not only is this pulsar the youngest of the 3000 known in our Milky Way galaxy, but it also belongs to a very rare category of pulsars: magnetars, the cosmic objects with the strongest magnetic fields ever measured in the Universe.
- “Swift J1818.0-1607 lies around 15,000 light-years away, within the Milky Way,” says lead author Paolo Esposito of the University School for Advanced Studies IUSS Pavia, Italy.
- “Spotting something so young, just after it formed in the Universe, is extremely exciting. People on Earth would have been able to see the supernova explosion that formed this baby magnetar around 240 years ago, right in the middle of the American and French revolutions.”
- The magnetar has yet more claims to fame. It is one of the fastest-spinning such objects known, whirling around once every 1.36 seconds – despite containing the mass of two Suns within a stellar remnant measuring just 25 km across.
- Immediately after the discovery, the astronomers looked at this object in further detail using XMM-Newton, NASA’s Swift and NuSTAR X-ray satellites, and the Sardinia Radio Telescope in Italy.

- Unlike most magnetars, which are only observable in X-rays, the observations revealed that Swift J1818.0-1607 is one of the very few to also show pulsed emission in radio waves.
- “Magnetars are fascinating objects, and this baby one appears to be especially intriguing given its extreme characteristics,” says Nanda Rea of the Institute of Space Sciences (CSIC, IEEC) in Barcelona, Spain, and principal investigator of the observations.
- “The fact that it can be seen in both radio waves and X-rays offers an important clue in an ongoing scientific debate on the nature of a specific type of stellar remnant: pulsars.”
- An especially magnetized type of pulsar, magnetars are generally thought to be uncommon in the Universe – astronomers have only detected around 30 – and are assumed to be distinct from other types of pulsar that show up strongly in radio emission.
- But X-ray researchers have long suspected that magnetars may be far more common than this view suggests. This new finding supports the idea that, rather than being exotic, they may instead form a substantial fraction of the pulsars found in the Milky Way.
- “The fact that a magnetar formed just recently indicates that this idea is well-founded,” explains co-author Alice Borghese, who worked on the data analysis with colleague Francesco Coti Zelati – both also based at the Institute of Space Sciences in Barcelona.
- “Astronomers have also discovered many magnetars in the past decade, doubling the known population,” she adds. “It’s likely that magnetars are just good at flying under the radar when they’re dormant, and are only discovered when they ‘wake up’ – as demonstrated by this baby magnetar, which was far less luminous before the outburst that led to its discovery.”
- Additionally, there may not be as wide a diversity of pulsars as initially thought. The distinctive phenomena shown by magnetars may also occur in other types of pulsar, just as Swift J1818.0-1607 exhibits characteristics – radio emission – not usually attributed to magnetars.
- “While interesting in their own right, magnetars are relevant on a far wider scale: they might play a key role in driving a whole host of transient events we see in the Universe,” adds Francesco.
- “Such events are thought to be somehow connected to magnetars either during their birth, or in the very early stages of their lives, making this discovery especially exciting.”

- Examples of transient events include gamma-ray bursts, super-luminous supernova explosions, and the mysterious fast radio bursts. These energetic events are potentially linked to the formation and existence of young, strongly magnetized objects – like Swift J1818.0-1607.
- “To infer this magnetar’s age, the researchers needed high-resolution long-term measurements of both the rate at which it is spinning, and of how this spin is changing over time,” adds ESA XMM-Newton Project Scientist Norbert Schartel.
- “XMM-Newton’s European Photon Imaging Camera, EPIC, observed Swift J1818.0−1607 just three days after it was discovered, enabling the researchers to extract an accurate picture of its X-ray emission, and characterize its rotation and spectral properties in detail.”
- “This kind of research is hugely important in understanding more about the stellar content of the Milky Way, and revealing the intricacies of phenomena occurring throughout the wider Universe.” 36)
• May 11, 2020: A new study, based on data from ESA’s XMM-Newton and NASA’s Chandra X-ray observatories, sheds new light on a three million light-year long bridge of hot gas linking two galaxy clusters, whose shape is being bent by the mighty activity of a nearby supermassive black hole. 37)
- Galaxy clusters are the largest objects in the Universe held together by gravity. They contain hundreds or thousands of galaxies, vast amounts of multi-million-degree gas that shines brightly in X-rays, and enormous reservoirs of unseen dark matter.
- The system portrayed in these images, called Abell 2384, is located 1.2 billion light years from Earth and comprises an overall mass of over 260 trillion times the mass of the Sun. In this case, the two galaxy clusters collided and then passed through each other, releasing a flood of hot gas from each cluster that formed an unusual bridge between the two objects.
- The X-ray view from XMM-Newton and Chandra is shown in blue, alongside observations in radio waves performed with the Giant Meterwave Radio Telescope in India (shown in red) and optical data from the Digitized Sky Survey (shown in yellow). The new multi-wavelength view reveals the effects of a jet shooting away from a supermassive black hole in the center of a galaxy in one of the clusters.




• April 8, 2020: Astronomers have assumed for decades that the Universe is expanding at the same rate in all directions. A new study based on data from ESA’s XMM-Newton, NASA’s Chandra and the German-led ROSAT X-ray observatories suggests this key premise of cosmology might be wrong. 38)
- Konstantinos Migkas, a PhD researcher in astronomy and astrophysics at the University of Bonn, Germany, and his supervisor Thomas Reiprich originally set out to verify a new method that would enable astronomers to test the so-called isotropy hypothesis. According to this assumption, the Universe has, despite some local differences, the same properties in each direction on the large scale.
- Widely accepted as a consequence of well-established fundamental physics, the hypothesis has been supported by observations of the cosmic microwave background (CMB). A direct remnant of the Big Bang, the CMB reflects the state of the Universe as it was in its infancy, at only 380,000 years of age. The CMB’s uniform distribution in the sky suggests that in those early days the Universe must have been expanding rapidly and at the same rate in all directions.
- In today’s Universe, however, this may no longer be true.

- The map shows the whole sky in the galactic coordinate system, with the center of our own galaxy, the Milky Way, located at the center of the map, and the plane of the galaxy – where most of its stars reside – oriented horizontally across the map (note that Milky Way stars are not shown in the map). The rate of the Universe’s expansion, indicated in terms of the so-called Hubble constant, is shown in different colors, with purple hues indicating a slower rate and orange/yellow hues indicating a faster rate.
- In a new study based on data from ESA’s XMM-Newton, NASA’s Chandra and the German-led ROSAT X-ray observatories, astronomers estimated the expansion rate using the X-ray temperature of hundreds of galaxy clusters across the sky and compared the temperature to the clusters’ brightnesses. Whereas they expected clusters of the same temperature and located at a similar distance to appear similarly bright, they noticed that clusters tended to be less bright than expected in one direction of the sky than in all others.
- The direction in the sky where galaxy clusters appeared less bright is represented by the region shown in purple in this map. If confirmed, the result might challenge the isotropy hypothesis, which assumes that the Universe has the same properties in each direction on large scales. This possibly uneven effect on cosmic expansion might be caused by the mysterious dark energy.
- “Together with colleagues from the University of Bonn and Harvard University, we looked at the behavior of over 800 galaxy clusters in the present Universe,” says Konstantinos. “If the isotropy hypothesis was correct, the properties of the clusters would be uniform across the sky. But we actually saw significant differences.”
- The astronomers used X-ray temperature measurements of the extremely hot gas that pervades the clusters and compared the data with how bright the clusters appear in the sky. Clusters of the same temperature and located at a similar distance should appear similarly bright. But that is not what the astronomers observed.
- “We saw that clusters with the same properties, with similar temperatures, appeared to be less bright than what we would expect in one direction of the sky, and brighter than expected in another direction,” says Thomas. “The difference was quite significant, around 30 per cent. These differences are not random but have a clear pattern depending on the direction in which we observed in the sky.”
- Before challenging the widely accepted cosmology model, which provides the basis for estimating the cluster distances, Konstantinos and colleagues first looked at other possible explanations. Perhaps, there could be undetected gas or dust clouds obscuring the view and making clusters in a certain area appear dimmer. The data, however, do not support this scenario.
- In some regions of space the distribution of clusters could be affected by bulk flows, large-scale motions of matter caused by the gravitational pull of extremely massive structures such as large cluster groups. This hypothesis, however, also seems unlikely. Konstantinos adds that the findings took the team by surprise.
- “If the Universe is truly anisotropic, even if only in the past few billion years, that would mean a huge paradigm shift because the direction of every object would have to be taken into account when we analyze their properties,” he says. “For example, today, we estimate the distance of very distant objects in the Universe by applying a set of cosmological parameters and equations. We believe that these parameters are the same everywhere. But if our conclusions are right than that would not be the case and we would have to revisit all our previous conclusions.”
- “This is a hugely fascinating result,” comments Norbert Schartel, XMM-Newton project scientist at ESA. “Previous studies have suggested that the present Universe might not be expanding evenly in all directions, but this result – the first time such a test has been performed with galaxy clusters in X-rays – has a much greater significance, and also reveals a great potential for future investigations.”
- The scientists speculate this possibly uneven effect on cosmic expansion might be caused by dark energy, the mysterious component of the cosmos which accounts for the majority – around 69% – of its overall energy. Very little is known about dark energy today, except that it appears to have been accelerating the expansion of the Universe in the past few billion years.

- The galaxies that belong to the cluster are concentrated towards the center, with two dominant members. Since galaxy clusters normally have only one major galaxy at their core, this suggests that XLSSC006 is undergoing a merger event.
- Pictured in this view, where the X-ray data are combined with a three-color composite of optical and near-infrared data from the Canada-France-Hawaii Telescope, are a multitude of other galaxies. Some are closer to us than the cluster – like the spiral galaxy towards the top right – and some are farther away. The image also shows a handful of foreground stars belonging to our Milky Way galaxy, which stand out with their diffraction spikes (a common artefact of astronomical images), while the small purple dots sprinkled across the frame are point sources of X-rays, many of them beyond the Milky Way.
- The X-ray data were obtained as part of the XXL Survey, XMM-Newton’s largest observational program to date, with follow-up observations performed by a number of other observatories around the world and in space. The latest XXL Survey release contains data for 365 galaxy clusters, tracing their large-scale distribution across cosmic history. These observations are helping astronomers refine our understanding of the Universe’s structure and evolution, and will serve as a reference for ESA’s future missions Euclid and Athena. 39)
• February 27, 2020: Astronomers using ESA’s XMM-Newton and NASA’s Chandra X-ray space observatories, along with radio telescopes on ground, have spotted the aftermath of the most powerful explosion ever seen in the Universe. 40)
- The huge outburst occurred in the Ophiuchus galaxy cluster, a large cosmic conglomerate with thousands of galaxies, hot gas and dark matter held together by gravity, lying some 390 million light years away. In particular, the eruption is linked to powerful jets released by the supermassive black hole that sits at the core of the cluster’s central galaxy and actively feeds on the surrounding gas, occasionally blasting off large amounts of matter and energy.
- The X-ray emission reveals the edge of a large cavity, carved in the hot gas by the black hole jets. The cavity is filled with radio emission from electrons accelerated to almost the speed of light – likely a result of the black hole’s feeding activity – providing evidence that an eruption of unprecedented size took place there.
![Figure 39: In this image, the diffuse hot gas pervading the cluster is revealed through X-ray observations from XMM-Newton (shown in pink), radio data from the Giant Meterwave Radio Telescope (shown in blue), and infrared data from the 2MASS survey (shown in white). Bright dots sprinkled across the image reflect the distribution of foreground stars and galaxies; in this complementary image, the inset in the lower right shows a zoomed-in X-ray view based on Chandra data (also shown in pink) [image credit: X-ray: ESA/XMM-Newton and NASA/CXC/Naval Research Lab/S. Giacintucci; Radio: NCRA/TIFR/GMRTN; Infrared: 2MASS/UMass/IPAC-Caltech/NASA/NSF]](https://www.eoportal.org/ftp/satellite-missions/x/XMM-Newton_040722/XMM-Newton_Auto66.jpeg)
- In 2016, a team of astronomers had found first hints of the giant explosion in the Chandra data, reporting the discovery of an unusual curved edge in the X-ray image of the Ophiuchus galaxy cluster. They considered whether this edge might point to a cavity in the hot gas linked to the black hole jets, but discarded the possibility at the time.
- In a more recent study, published in February 2020, Simona Giacintucci of the Naval Research Laboratory in Washington, DC (US) and collaborators detected the curved edge also in XMM-Newton data, confirming the earlier Chandra observation. In this study, the scientists combined the X-ray data with radio wave observations of the Ophiuchus cluster from the Murchison Widefield Array (MWA) in Australia and the Giant Meterwave Radio Telescope (GMRT) in India, revealing that the curved edge delimits a region filled with radio-emitting gas and is indeed part of the wall of a cavity in the hot gas.
- The black hole eruption that created the cavity released an amount of energy about five times greater than that involved in the most powerful event of this type known to date, observed in the galaxy cluster MS0735.6+7421.
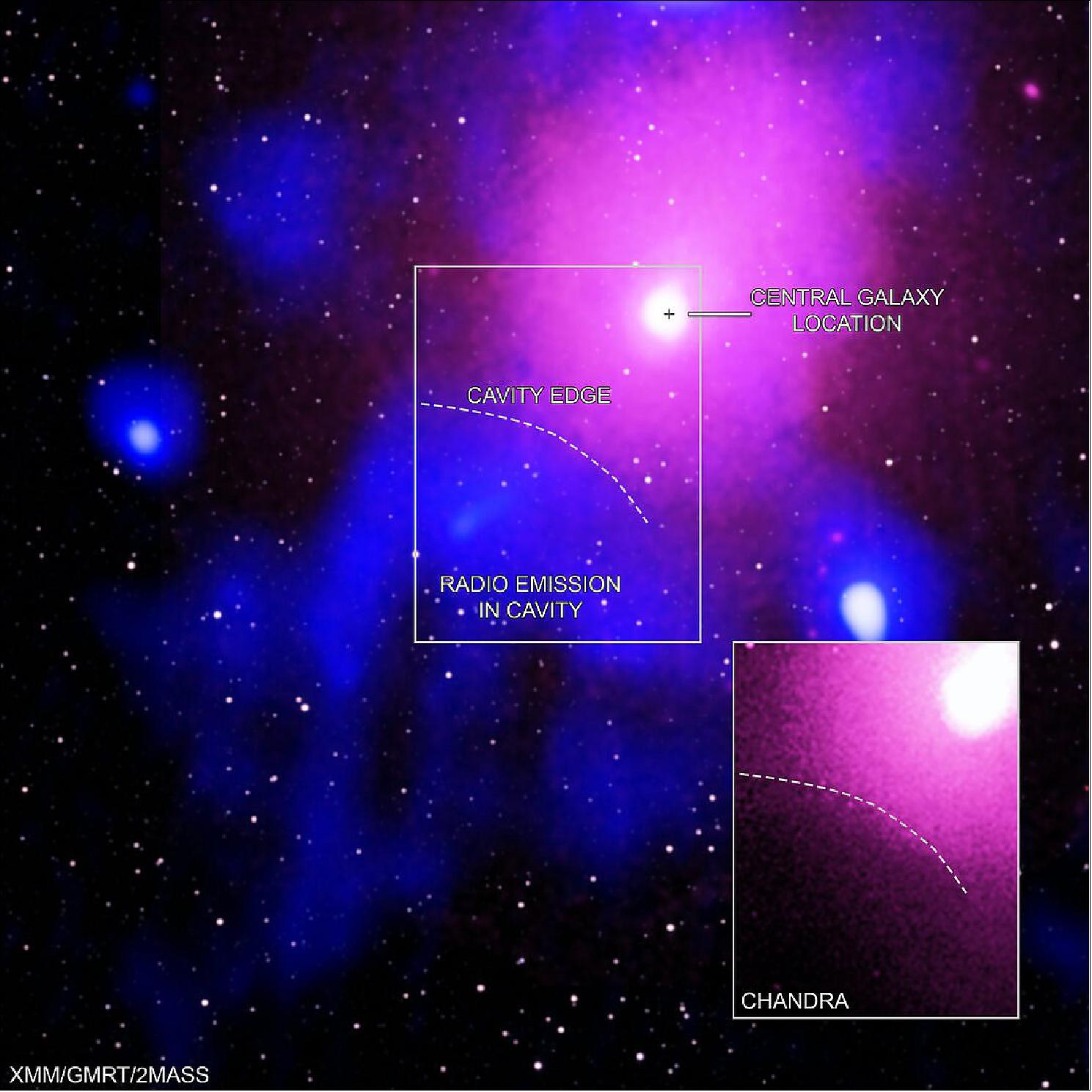
• February 20, 2020: A star of about eight percent the Sun’s mass has been caught emitting an enormous ‘super flare’ of X-rays – a dramatic high-energy eruption that poses a fundamental problem for astronomers, who did not think it possible on stars that small. 41)
- The culprit, known by its catalog number J0331-27, is a kind of star called an L dwarf. This is a star with so little mass that it is only just above the boundary of actually being a star. If it had any less mass, it would not possess the internal conditions necessary to generate its own energy.
- Astronomers spotted the enormous X-ray flare in data recorded on 5 July 2008 by the European Photon Imaging Camera (EPIC) onboard ESA’s XMM-Newton X-ray observatory. In a matter of minutes, the tiny star released more than ten times more energy of even the most intense flares suffered by the Sun.
- Flares are released when the magnetic field in a star’s atmosphere becomes unstable and collapses into a simpler configuration. In the process, it releases a large proportion of the energy that has been stored in it.
- This explosive release of energy creates a sudden brightening – the flare – and this is where the new observations present their biggest puzzle.
- “This is the most interesting scientific part of the discovery, because we did not expect L-dwarf stars to store enough energy in their magnetic fields to give rise to such outbursts,” says Beate Stelzer, Institut für Astronomie und Astrophysik Tübingen, Germany, and INAF – Osservatorio Astronomico di Palermo, Italy, who was part of the study team. 42)
- Energy can only be placed in a star’s magnetic field by charged particles, which are also known as ionized material and created in high-temperature environments. As an L dwarf, however, J0331-27 has a low surface temperature for a star – just 2100 K compared to the roughly 6000 K on the Sun. Astronomers did not think such a low temperature would be capable of generating enough charged particles to feed so much energy into the magnetic field. So the conundrum is: how a super flare is even possible on such a star.
- “That’s a good question,” says Beate. “We just don’t know – nobody knows.”
- The super flare was discovered in the XMM-Newton data archive as part of a large research project led by Andrea De Luca of INAF – Istituto di Astrofisica Spaziale e Fisica Cosmica in Milan, Italy. The project studied the temporal variability of around 400,000 sources detected by XMM-Newton over 13 years.
- Andrea and collaborators were particularly looking for peculiar phenomena and in J0331-27 they certainly got that. A number of similar stars had been seen to emit super flares in the optical part of the spectrum, but this is the first unambiguous detection of such an eruption at X-ray wavelengths.
- The wavelength is significant because it signals which part of the atmosphere the super flare is coming from: optical light comes from deeper in the star’s atmosphere, near its visible surface, whereas X-rays come from higher up in the atmosphere.
- Understanding the similarities and differences between this new – and so far unique – super flare on the L dwarf and previously observed flares, detected at all wavelengths on stars of higher mass is now a priority for the team. But to do that, they need to find more examples.
- “There is still much to be discovered in the XMM-Newton archive,” says Andrea. “In a sense, I think this is only the tip of the iceberg.”
- One clue they do have is that there is only one flare from J0331-27 in the data, despite XMM-Newton having observed the star for a total of 3.5 million seconds – about 40 days. This is peculiar because other flaring stars tend to suffer from numerous smaller flares too.
- “The data seem to imply that it takes an L dwarf longer to build up the energy, and then there is one sudden big release,” says Beate.
- Stars that flare more frequently release less energy each time, while this L dwarf seems to release energy very rarely but then in a really big event. Why this might be the case is still an open question that needs further investigation.

- “The discovery of this L dwarf super flare is a great example of research based on the XMM-Newton archive, demonstrating the mission's enormous scientific potential,” says Norbert Schartel, XMM Newton project scientist for ESA. “I look forward to the next surprise.”
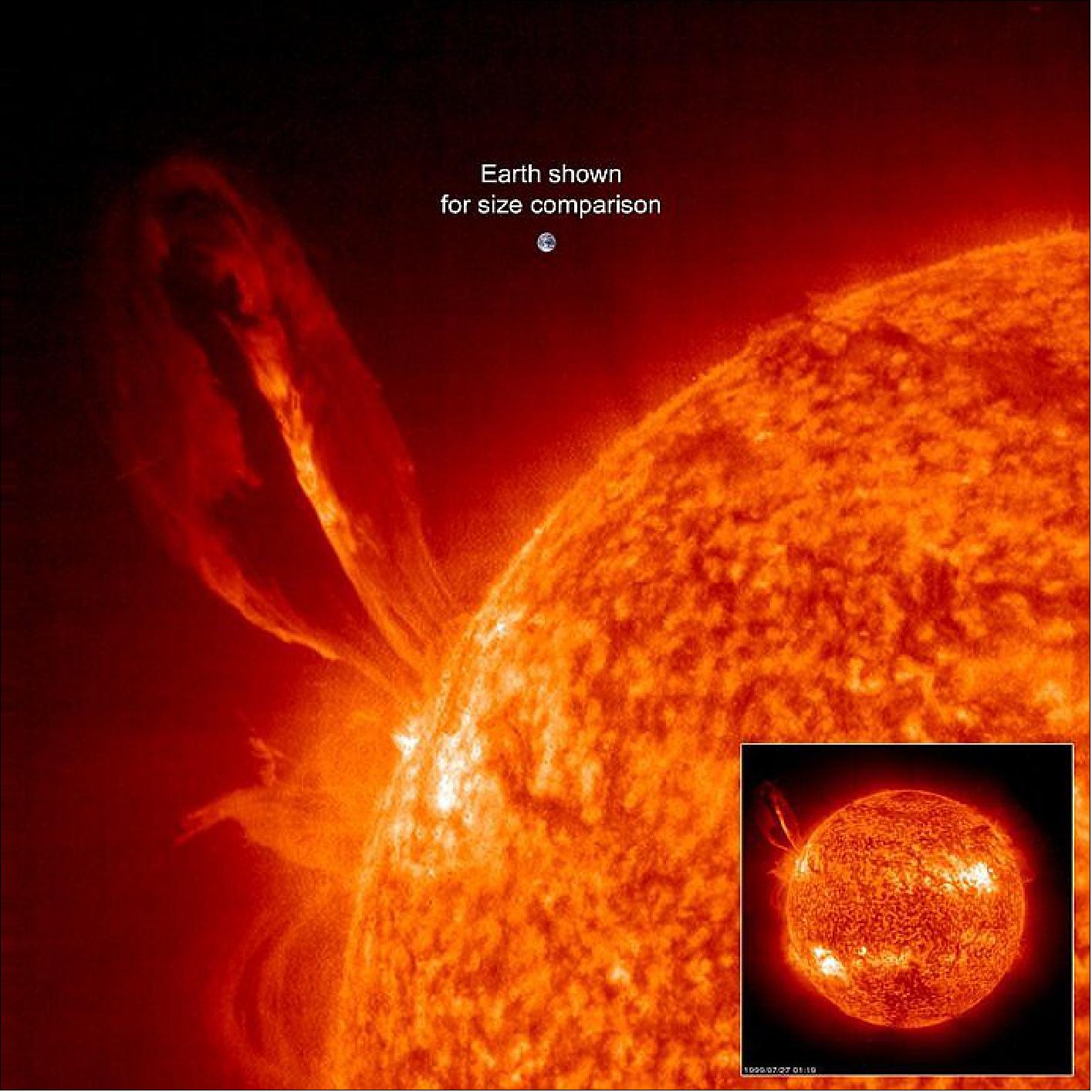
• January 20, 2020: Material falling into a black hole casts X-rays out into space – and now, for the first time, ESA’s XMM-Newton X-ray observatory has used the reverberating echoes of this radiation to map the dynamic behavior and surroundings of a black hole itself. 43)
- Most black holes are too small on the sky for us to resolve their immediate environment, but we can still explore these mysterious objects by watching how matter behaves as it nears, and falls into, them.
- As material spirals towards a black hole, it is heated up and emits X-rays that, in turn, echo and reverberate as they interact with nearby gas. These regions of space are highly distorted and warped due to the extreme nature and crushingly strong gravity of the black hole.
- For the first time, researchers have used XMM-Newton to track these light echoes and map the surroundings of the black hole at the core of an active galaxy. Named IRAS 13224–3809, the black hole’s host galaxy is one of the most variable X-ray sources in the sky, undergoing very large and rapid fluctuations in brightness of a factor of 50 in mere hours.
- “Everyone is familiar with how the echo of their voice sounds different when speaking in a classroom compared to a cathedral – this is simply due to the geometry and materials of the rooms, which causes sound to behave and bounce around differently,” explains William Alston of the University of Cambridge, UK, lead author of the new study. 44)
- “In a similar manner, we can watch how echoes of X-ray radiation propagate in the vicinity of a black hole in order to map out the geometry of a region and the state of a clump of matter before it disappears into the singularity. It’s a bit like cosmic echo-location.”

- As the dynamics of infalling gas are strongly linked to the properties of the consuming black hole, William and colleagues were also able to determine the mass and spin of the galaxy’s central black hole by observing the properties of matter as it spiralled inwards.
- The inspiralling material forms a disc as it falls into the black hole. Above this disc lies a region of very hot electrons – with temperatures of around a billion degrees – called the corona. While the scientists expected to see the reverberation echoes they used to map the region’s geometry, they also spotted something unexpected: the corona itself changed in size incredibly quickly, over a matter of days.
- “As the corona’s size changes, so does the light echo – a bit like if the cathedral ceiling is moving up and down, changing how the echo of your voice sounds,” adds William.
- “By tracking the light echoes, we were able to track this changing corona, and – what’s even more exciting – get much better values for the black hole’s mass and spin than we could have determined if the corona was not changing in size. We know the black hole's mass cannot be fluctuating, so any changes in the echo must be down to the gaseous environment.”
- The study used the longest observation of an accreting black hole ever taken with XMM-Newton, collected over 16 spacecraft orbits in 2011 and 2016 and totalling 2 million seconds – just over 23 days.
- This, combined with the strong and short-term variability of the black hole itself, allowed William and collaborators to model the echoes comprehensively over day-long timescales.
- The region explored in this study is not accessible to observatories such as the Event Horizon Telescope, which managed to take the first ever picture of gas in the immediate vicinity of a black hole – the one sitting at the center of the nearby massive galaxy M87. The result, based on observations performed with radio telescopes across the world in 2017 and published last year, immediately became a global sensation.
- “The Event Horizon Telescope image was obtained using a method known as interferometry – a wonderful technique that can only work on the very few nearest supermassive black holes to Earth, such as those in M87 and in our home galaxy, the Milky Way, because their apparent size on the sky is large enough for this method to work,” says co-author Michael Parker, who is an ESA research fellow at the European Space Astronomy Center near Madrid, Spain.
- “By contrast, our approach is able to probe the nearest few hundred supermassive black holes that are actively consuming matter – and this number will increase significantly with the launch of ESA’s Athena satellite.”
- Characterizing the environments closely surrounding black holes is a core science goal for ESA’s Athena mission, which is scheduled for launch in the early 2030s and will unveil the secrets of the hot and energetic Universe.
- Measuring the mass, spin and accretion rates of a large sample of black holes is key to understanding gravity throughout the cosmos.
- Additionally, since supermassive black holes are strongly linked to their host galaxy’s properties, these studies are also key to furthering our knowledge of how galaxies form and evolve over time.
- “The large dataset provided by XMM-Newton was essential for this result,” says Norbert Schartel, ESA XMM-Newton Project Scientist.
- “Reverberation mapping is an exciting technique that promises to reveal much about both black holes and the wider Universe in coming years. I hope that XMM-Newton will perform similar observing campaigns for several more active galaxies in coming years, so that the method is fully established when Athena launches.”

• January 16, 2020: ESA’s XMM-Newton has discovered that gas lurking within the Milky Way’s halo reaches far hotter temperatures than previously thought and has a different chemical make-up than predicted, challenging our understanding of our galactic home. 46)
- A halo is a vast region of gas, stars and invisible dark matter surrounding a galaxy. It is a key component of a galaxy, connecting it to wider intergalactic space, and is thus thought to play an important role in galactic evolution.
- Until now, a galaxy’s halo was thought to contain hot gas at a single temperature, with the exact temperature of this gas dependent on the mass of the galaxy.
- However, a new study using ESA’s XMM-Newton X-ray space observatory now shows that the Milky Way’s halo contains not one but three different components of hot gas, with the hottest of these being a factor of ten hotter than previously thought. This is the first time multiple gas components structured in this way have been discovered in not only the Milky Way, but in any galaxy. 47)
- “We thought that gas temperatures in galactic haloes ranged from around 10,000 to one million degrees – but it turns out that some of the gas in the Milky Way’s halo can hit a scorching 10 million degrees,” says Sanskriti Das, a graduate student at The Ohio State University, USA, and lead author of the new study.
- “While we think that gas gets heated to around one million degrees as a galaxy initially forms, we’re not sure how this component got so hot. It may be due to winds emanating from the disc of stars within the Milky Way.”
- The study used a combination of two instruments aboard XMM-Newton: the Reflection Grating Spectrometer (RGS) and European Photon Imaging Camera (EPIC). EPIC was used to study the light emitted by the halo, and RGS to study how the halo affects and absorbs light that passes through it.
- To probe the Milky Way’s halo in absorption, Sanskriti and colleagues observed an object known as a blazar: the very active, energetic core of a distant galaxy that is emitting intense beams of light.
- Having travelled almost five billion light-years across the cosmos, the X-ray light from this blazar also passed through our galaxy’s halo before reaching XMM-Newton’s detectors, and thus holds clues about the properties of this gaseous region.
- Unlike previous X-ray studies of the Milky Way’s halo, which normally last a day or two, the team performed observations over a period of three weeks, enabling them to detect signals that are usually too faint to see.
- “We analyzed the blazar’s light and zeroed in on its individual spectral signatures: the characteristics of the light that can tell us about the material it’s passed through on its way to us,” says co-author Smita Mathur, also of The Ohio State University, and Sanskriti’s advisor.
- “There are specific signatures that only exist at specific temperatures, so we were able to determine how hot the halo gas must have been to affect the blazar light as it did.”
- The Milky Way’s hot halo is also significantly enhanced with elements heavier than helium, which are usually produced in the later stages of a star’s life. This indicates that the halo has received material created by certain stars during their lifetimes and final stages, and flung out into space as they die.
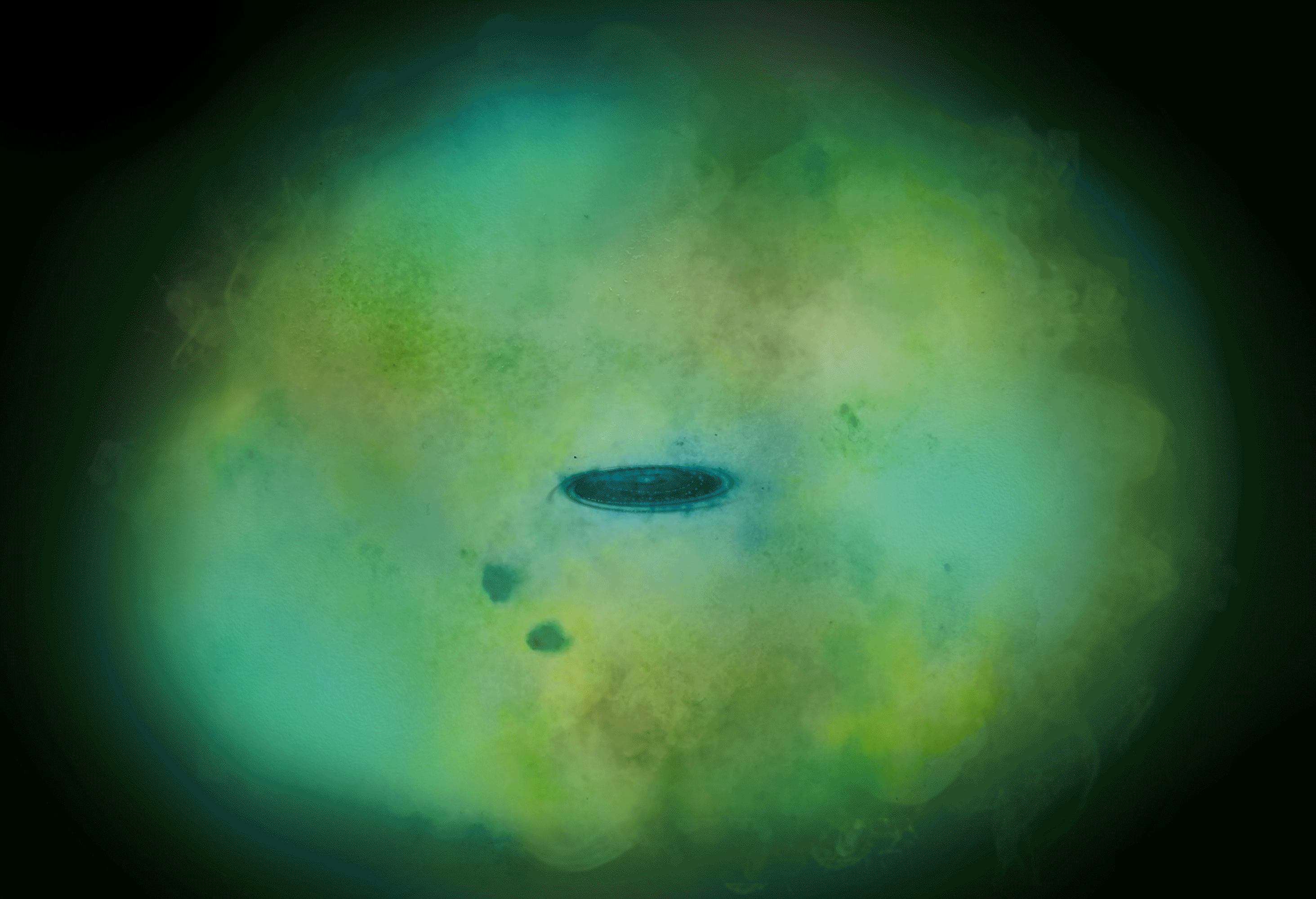
- A study using XMM-Newton now shows that the Milky Way’s halo contains not one but three different components of hot gas, with the hottest of these being a factor of ten hotter than previously thought. This is the first time multiple gas components structured in this way have been discovered in not only the Milky Way, but in any galaxy.
- A study using XMM-Newton now shows that the Milky Way’s halo contains not one but three different components of hot gas, with the hottest of these being a factor of ten hotter than previously thought. This is the first time multiple gas components structured in this way have been discovered in not only the Milky Way, but in any galaxy.
- “Until now, scientists have primarily looked for oxygen, as it’s abundant and thus easier to find than other elements,” explains Sanskriti.
- “Our study was more detailed: we looked at not only oxygen but also nitrogen, neon and iron, and found some hugely interesting results.”
- Scientists expect the halo to contain elements in similar ratios to those seen in the Sun. However, Das and colleagues noticed less iron in the halo than expected, indicating that the halo has been enriched by massive dying stars, and also less oxygen, likely due to this element being taken up by dusty particles in the halo.
- “This is really exciting – it was completely unexpected, and tells us that we have much to learn about how the Milky Way has evolved into the galaxy it is today,” adds Sanskriti.

- The newly discovered hot gas component also has wider implications that affect our overall understanding of the cosmos. Our galaxy contains far less mass than we expect: this is known as the ‘missing matter problem’, in that what we observe does not match up with theoretical predictions.
- From its long-term mapping of the cosmos, ESA’s Planck spacecraft predicted that just under 5% of the mass in the Universe should exist in the form of ‘normal’ matter – the kind making up stars, galaxies, planets, and so on.
- “However, when we add up everything we see, our figure is nowhere near this prediction,” adds co-author Fabrizio Nicastro of Osservatorio Astronomico di Roma—INAF, Italy, and the Harvard-Smithsonian Center for Astrophysics, USA.
- “So where’s the rest? Some suggest that it may be hiding in the extended and massive halos surrounding galaxies, making our finding really exciting.”
- As this hot component of the Milky Way’s halo has never been seen before, it may have been overlooked in previous analyses – and may thus contain a large amount of this ‘missing’ matter.
- “These observations provide new insights into the thermal and chemical history of the Milky Way and its halo, and challenge our knowledge of how galaxies form and evolve,” concludes ESA XMM project scientist Norbert Schartel.
- “The study looked at the halo along one sightline – that towards the blazar – so it will be hugely exciting to see future research expand on this.”
• January 10, 2020: Using XMM-Newton to study Perseus, astronomers spotted the first signs of this hot gas splashing and sloshing around – a behavior that, while predicted, had never been seen before. 48)
- ESA’s XMM-Newton X-ray observatory has spied hot gas sloshing around within a galaxy cluster – a never-before-seen behavior that may be driven by turbulent merger events.
- Galaxy clusters are the largest systems in the Universe bound together by gravity. They contain hundreds to thousands of galaxies and large quantities of hot gas known as plasma, which reaches temperatures of around 50 million degrees and shines brightly in X-rays.
- Very little is known about how this plasma moves, but exploring its motions may be key to understanding how galaxy clusters form, evolve and behave.
- “We selected two nearby, massive, bright and well-observed galaxy clusters, Perseus and Coma, and mapped how their plasma moved – whether it was moving towards or away from us, its speed, and so on – for the first time,” says Jeremy Sanders of the Max Planck Institute for Extraterrestrial Physics in Garching, Germany, and lead author of the new study. 49)
- “We did this over large regions of sky: an area roughly the size of two full Moons for Perseus, and four for Coma. We really needed XMM-Newton for this, as it’d be extremely difficult to cover such large areas with any other spacecraft.”
- Jeremy and colleagues found direct signs of plasma flowing, splashing and sloshing around within the Perseus galaxy cluster – one of the most massive known objects in the Universe, and the brightest cluster in the sky in terms of X-rays. While this kind of motion has been predicted theoretically, it had never been seen before in the cosmos.
- By looking at simulations of how the plasma moved within the cluster, the researchers then explored what was causing the sloshing. They found it to be likely due to smaller sub-clusters of galaxies colliding and merging with the main cluster itself. These events are energetic enough to disrupt Perseus’ gravitational field and kickstart a sloshing motion that will last for many millions of years before settling.



- Unlike Perseus, which is characterized by a main cluster and several smaller sub-structures, the Coma cluster contained no sloshing plasma, and appears to instead be a massive cluster made up of two major sub-clusters that are slowly merging together.
- “Coma contains two massive central galaxies rather than a cluster’s usual single behemoth, and different regions appear to contain material that moves differently,” says Jeremy.
- “This indicates that there are multiple streams of material within the Coma cluster that haven’t yet come together to form a single coherent ‘blob’, like we see with Perseus.”
- The finding was made possible by a new calibration technique applied to XMM-Newton’s European Photon Imaging Camera (EPIC). The ingenious method, which involved mining two decades of archival EPIC data, improved the accuracy of the camera’s velocity measurements by a factor of over 3.5, raising XMM-Newton’s capabilities to a new level.
- “The EPIC camera has an instrumental background signal – the so-called ‘fluorescent lines’ which are always present in our data, and can sometimes be annoying as they’re usually not what we’re looking for,” adds co-author Ciro Pinto, an ESA research fellow at ESTEC (European Space Research and Technology Center) in Noordwijk, The Netherlands, who recently moved to Italy’s National Institute for Astrophysics.
- “We decided to use these lines, which are a constant feature, to compare and align EPIC data from the past 20 years to better determine how the camera behaves, and then used this to correct for any instrumental variation or effects.”
- This technique made it possible to map the gas in the clusters more accurately. Jeremy, Ciro and colleagues used the background lines to recognize and remove individual variations between observations, and then eliminated any subtler instrumental effects identified and flagged up by their 20 years of EPIC data mining.
- EPIC comprises three CCD cameras designed to capture both low- and high-energy X-rays, and is one of a trio of advanced instruments aboard XMM-Newton.

- Exploring the dynamic X-ray sky since its launch in 1999, XMM-Newton is the biggest scientific satellite ever built in Europe, and carries some of the most powerful telescope mirrors ever developed.
- “This calibration technique highlights newfound capabilities of the EPIC camera,” says Norbert Schartel, ESA XMM-Newton Project Scientist.
- “High-energy astrophysics often entails comparing X-ray data at different points in the cosmos for everything from plasma to black holes, so the ability to minimize instrumental effects is key. By using past XMM-Newton observations to refine future ones, the new technique may open up inspiring opportunities for new research and discovery.”
- These XMM-Newton observations will also remain unparalleled until the launch of ESA’s Advanced Telescope for High-ENergy Astrophysics (ATHENA) in 2031. Whereas covering such large areas of sky will largely be beyond the capabilities of telescopes such as the upcoming JAXA/NASA XRISM (X-ray Imaging and Spectroscopy Mission), Athena will combine a large X-ray telescope with state-of-the-art scientific instruments to shed new light on the hot, energetic Universe.
• On 10 December 2019, ESA’s XMM-Newton X-ray space observatory is celebrating its 20th launch anniversary. In those two decades, the observatory has supplied a constant stream of outstanding science. One area that the mission has excelled in is the science of black holes, having had a profound effect on our understanding of these cosmic enigmas. 50)

- Although neither XMM-Newton nor any other telescope can actually see black holes in this detail, the mission’s data and observations have provided a great source of information about these mysterious gravitational traps. In particular, XMM-Newton has been particularly good at isolating the X-rays given out by high-temperature, ionized atoms of iron as they swirl towards doom in the black hole.
- The X-rays given out from the iron contain information about the geometry and dynamics of the black hole. In 2013, XMM-Newton was used to measure such emission in order to study the rotation rate of the supermassive black hole at the center of the spiral galaxy NGC 1365.
- Supermassive black holes, with masses between millions and billions of times the mass of our Sun, are thought to lurk in the center of almost every large galaxy in the Universe. Their rotation rate is important because it can give away important details about the history of their host galaxy.
- A fast rotating black hole is fed by a uniform stream of matter falling together, or by galaxies merging with one another, whereas a slowly rotating black hole is buffeted from all sides by small clumps of matter hitting it. In the case of NGC 1365, XMM-Newton showed that the black hole was rotating quickly and so the galaxy probably grew steadily over time, or merged with others.
- More recently, XMM-Newton discovered mysterious flashes from the black hole at the center of another galaxy called GSN 069. These flares took place every nine hours or so, raising the brightness of the X-ray emission by a factor of 100. These eruptions are thought to be coming from the matter caught in the black hole’s gravitational grip or from a less massive black hole circling the more massive one.
- As XMM-Newton continues into its third decade, black holes and the galaxies they are found in will continue to be a priority target.
• September 11, 2019: ESA’s X-ray space telescope XMM-Newton has detected never-before-seen periodic flares of X-ray radiation coming from a distant galaxy that could help explain some enigmatic behaviors of active black holes. 51)
- XMM-Newton, the most powerful X-ray observatory, discovered some mysterious flashes from the active black hole at the core of the galaxy GSN 069, about 250 million light years away. On 24 December 2018, the source was seen to suddenly increase its brightness by up to a factor 100, then dimmed back to its normal levels within one hour and lit up again nine hours later.
![Figure 52: An X-ray view of the active black hole at the core of distant galaxy GSN 069, about 250 million light years away, based on data from ESA’s XMM-Newton X-ray observatory. The upper part of the animation shows the actual observations, and the graph in the lower part shows variations of the X-ray brightness of the source relative to its ‘dormant’ level. - This animation is based on nearly 40 hours of observations of this source, which undergoes never-before-seen flashes – dubbed QPEs (Quasi-Periodic Eruptions) every nine hours. The sequence has been speeded up for illustration purposes; each frame corresponds to about three minutes of actual XMM-Newton exposure time [image credit: ESA/XMM-Newton; G. Miniutti & M. Giustini (CAB, CSIC-INTA, Spain)]](https://www.eoportal.org/ftp/satellite-missions/x/XMM-Newton_040722/XMMBlackhole.gif)
- “It was completely unexpected,” says Giovanni Miniutti, of the Centro de Astrobiología in Madrid, Spain, lead author of a new paper published in the journal Nature today. 52)
- “Giant black holes regularly flicker like a candle but the rapid, repeating changes seen in GSN 069 from December onwards are something completely new.”
- Further observations, performed with XMM-Newton as well as NASA’s Chandra X-ray observatory in the following couple of months, confirmed that the distant black hole was still keeping the tempo, emitting nearly periodic bursts of X-rays every nine hours. The researchers are calling the new phenomenon QPEs.
- “The X-ray emission comes from material that is being accreted into the black hole and heats up in the process,” explains Giovanni.
- “There are various mechanisms in the accretion disc that could give rise to this type of quasi-periodic signal, potentially linked to instabilities in the accretion flow close to the central black hole.
- “Alternatively, the eruptions could be due to the interaction of the disc material with a second body – another black hole or perhaps the remnant of a star previously disrupted by the black hole.”
- Although never before observed, Giovanni and colleagues think periodic flares like these might actually be quite common in the Universe.

- It is possible that the phenomenon had not been identified before because most black holes at the cores of distant galaxies, with masses millions to billions of times the mass of our Sun, are much larger than the one in GSN 069, which is only about 400,000 times more massive than our Sun.
- The bigger and more massive the black hole, the slower the fluctuations in brightness it can display, so a typical supermassive black hole would erupt not every nine hours, but every few months or years. This would make detection unlikely as observations rarely span such long periods of time.
- And there is more. Quasi-periodic eruptions like those found in GSN 069 could provide a natural framework to interpret some puzzling patterns observed in a significant fraction of active black holes, whose brightness seems to vary too fast to be easily explained by current theoretical models.
- “We know of many massive black holes whose brightness rises or decays by very large factors within days or months, while we would expect them to vary at a much slower pace,” says Giovanni.
- “But if some of this variability corresponds to the rise or decay phases of eruptions similar to those discovered in GSN 069, then the fast variability of these systems, which appears currently unfeasible, could naturally be accounted for. New data and further studies will tell if this analogy really holds.”
- The quasi-periodic eruptions spotted in GSN 069 could also explain another intriguing property observed in the X-ray emission from nearly all bright, accreting supermassive black holes: the so-called ‘soft excess’.
![Figure 54: X-ray flares from active galaxy GSN 069. Variations in the X-ray brightness of the active black hole at the core of distant galaxy GSN 069, about 250 million light years away, as recorded by ESA’s XMM-Newton X-ray observatory (blue) and NASA’s Chandra X-ray observatory (red). The graph shows the X-ray brightness of the source relative to its ‘dormant’ level. This source was first observed to undergo before-seen flashes on 24 December 2018, when its brightness suddenly increased by up to a factor 100, then dimmed back to its normal levels within one hour and lit up again nine hours later. Further observations performed over a period of 54 days confirmed this flaring behavior, with ‘quasi-periodic eruptions’, or QPEs detected every nine hours [image credit: ESA/XMM-Newton; NASA/CXC; G. Miniutti (CAB, CSIC-INTA, Spain)]](https://www.eoportal.org/ftp/satellite-missions/x/XMM-Newton_040722/XMM-Newton_Auto5B.jpeg)
- It consists in enhanced emission at low X-ray energies, and there is still no consensus on what causes it, with one leading theory invoking a cloud of electrons heated up near the accretion disc.
- Like similar black holes, GSN 069 exhibits such a soft X-ray excess during bursts, but not between eruptions.
- “We may be witnessing the formation of the soft excess in real time, which could shed light on its physical origin,” says co-author Richard Saxton from the XMM-Newton operation team at ESA’s astronomy center in Spain.
- “How the cloud of electrons is created is currently unclear, but we are trying to identify the mechanism by studying the changes in the X-ray spectrum of GSN 069 during the eruptions.”
- The team is already trying to pinpoint the defining properties of GSN 069 at the time when the periodic eruptions were first detected to look for more cases to study.
- "One of our immediate goals is to search for X-ray quasi-periodic eruptions in other galaxies, to further understand the physical origin of this new phenomenon,” adds co-author Margherita Giustini of Madrid’s Centro de Astrobiología.
- “GSN 069 is an extremely fascinating source, with the potential to become a reference in the field of black hole accretion,” says Norbert Schartel, ESA’s XMM-Newton project scientist.
- The discovery would not have been possible without XMM-Newton’s capabilities.
- “These bursts happen in the low energy part of the X-ray band, where XMM-Newton is unbeatable. We will certainly need to use the observatory again if we want to find more of these kinds of events in the future,” concludes Norbert.
• August 26, 2019: This colorful spread of light specks is in fact a record of extremely powerful phenomena taking place in a galaxy known as Messier 83, or M83. Located some 15 million light-years away, M83 is a barred spiral galaxy, not dissimilar in shape from our own Milky Way, and currently undergoing a spur of star formation, with a handful of new stars being born every year. 53)

- Most of the dots in this view represent the end points of the life cycle of stars, including remnants of supernova explosions and binary systems featuring compact stellar remnants like neutron stars or black holes that are feeding on matter from a companion star. In particular, the large speck to the lower left of the galaxy’s central region is what astronomers call an ultra-luminous X-ray source, or ULX, a binary system where the compact remnant is accreting mass from its companion at a much higher rate than an ordinary X-ray binary.
- The highly energetic phenomena that can be observed with X-ray telescopes often undergo regular changes, on time scales of days or even hours, turning the X-ray sky into a spectacular light show.
- The sources located in the reddish area at the center of the image correspond to objects located in the inner portions of M83. The majority of sources scattered across the image are located in the outskirts of the galaxy, but a few of those are foreground stars in our own galaxy, and others correspond to more distant galaxies in the background. 54)
• July 8, 2019: While observing the sky in X-rays, ESA’s XMM-Newton spots thousands and thousands of serendipitous sources. The catalog, released in May 2018, features sources in the 0.2 to 12 keV energy range drawn from 10,242 observations made by XMM-Newton’s European Photon Imaging Camera (EPIC), an instrument capable of detecting very faint sources and rapid changes in intensity, between 3 February 2000 and 30 November 2017. It contains 532 more observations and 47,363 more detections than the preceding 3XMM-DR7 catalog, which was made public in June 2017. 55)
- While the pattern of sources across the sky may appear random, some structure can be seen here. The oval represents the celestial sphere, an abstract perspective upon which our observations of the Universe are projected. The data are plotted in galactic coordinates, such that the center of the plot corresponds to the center of our Milky Way galaxy – and this can be seen in the image. Through the center of the oval is a horizontal line, where patches of purple appear to draw together. This line is the plane of the Milky Way galaxy, with the large splotch of color in the center corresponding to our galaxy’s core, where XMM-Newton made a higher number of serendipitous detections.
- XMM-Newton has been orbiting the Earth since 1999, observing the cosmos around us while on the hunt for X-rays coming from high-energy phenomena such as black holes, stellar winds, pulsars, and neutron stars. With every patch of sky that XMM-Newton observes, the telescope detects between 50 and 100 serendipitous sources, such as those shown here, besides the objects that were the original target of the observations. This is due to the large collecting area of the telescope’s mirrors and its wide field of view.
- All-sky images and large-scale cosmic data are immensely valuable in our study of the cosmos. Upcoming missions – such as the eROSITA space telescope, a German-led satellite scheduled for launch on 12 July 2019 to complete the first all-sky survey in the medium-energy X-ray band, up to 10 keV – will add to this wealth of knowledge, and help further our understanding of the X-ray Universe.
• May 6, 2019: The image of Figure 57 shows a quasar nicknamed the Teacup due to its shape. A quasar is an active galaxy that is powered by material falling into its central supermassive black hole. They are extremely luminous objects located at great distances from Earth. The Teacup is 1.1 billion light years away and was thought to be a dying quasar until recent X-ray observations shed new light on it. 56) 57)
- The Teacup was discovered in 2007 as part of the Galaxy Zoo project, a citizen science project that classified galaxies using data from the Sloan Digital Sky Survey. A powerful eruption of energy and particles from the central black hole created a bubble of material that became the Teacup's handle, which lies around 30,000 light years from the center.
- Observations revealed ionized atoms in the handle of the Teacup, possibly caused by strong radiation coming from the quasar in the past. This past level of radiation dwarfed the current measurements of the luminosity from the quasar. The radiation seemed to have diminished by 50 to 600 times over the last 40,000 to 100,000 years, leading to the theory that the quasar was rapidly fading.
- But new data from ESA's XMM-Newton telescope and NASA's Chandra X-ray observatory reveal that X-rays are coming from a heavily obscured central source, which suggests that the quasar is still burning bright beneath its shroud. While the quasar has certainly dimmed over time, it is nowhere near as significant as originally thought, perhaps only fading by a factor of 25 or less over the past 100,000 years.
- The Chandra data also showed evidence for hotter gas within the central bubble, and close to the 'cup' which surrounds the central black hole. This suggests that a wind of material is blowing away from the black hole, creating the teacup shape.

• April 10, 2019: A giant elliptical galaxy, M87 is home to several trillion stars, making it one of the most massive galaxies in the local Universe. About 52 million light years away, it is located at the center of the Virgo cluster, the nearest cluster of galaxies to the Local Group, to which our own Milky Way galaxy belongs. 58)
- A supermassive black hole as massive as billions of stars like our Sun sits at the core of M87, accreting material from its surroundings at an extremely intense rate. The black hole’s accretion produces powerful jets that launch energetic particles close to the speed of light outwards into the surrounding cluster environment, as well as inflating giant bubbles that lift cooler gas from the cluster center and form the filamentary structures visible in this image.
- On 10 April 2019, the EHT (Event Horizon Telescope) – a planet-scale array of eight ground-based radio telescopes forged through international collaboration – presented the first direct visual evidence of a supermassive black hole and its shadow: the black hole at the core of M87. The EHT observations were also performed in 2017.

• April 8, 2019: An X-ray machine which uses space technology to generate crystal clear images that doctors can use to detect the early signs of cancer has been prioritized for €1.2 m of funding by the European Space Agency and the UK Space Agency. 59)
- Cancers are often missed on normal X-rays, which produce slightly fuzzy images that can be difficult to interpret. This can mean the disease is more advanced and difficult to treat by the time it is discovered.

- So engineers from the UK company Adaptix have used technology developed for space to produce three-dimensional scans that generate much clearer images.
- The device employs X-ray optics deployed on spacecraft such as ESA’s XMM-Newton mission, which launched in 1999 and is observing stars at X-ray wavelengths.
- Miniaturized, portable and connected through satellites, the machine should also allow patients to be scanned in GPs’ surgeries, reducing the need for trips to hospital and shortening waiting times for patients.
- Tony Young, national clinical director for innovation at NHS (National Health Service) England, said: “Last year as we celebrated the NHS’s 70th birthday, we challenged industry to bring technology designed for outer space into the NHS. Using stargazing technology to spot cancer is exactly the type of advanced innovation that could improve care for patients by speeding up diagnosis and helping to deliver our long-term plan which will save half a million lives.”
- Chris Skidmore, the UK science minister, said: “The challenge of working in space focuses some of the UK’s most brilliant minds. These experts can also help transform our lives for the better here on Earth.
- “The huge potential of space technology isn’t just about reaching out into the universe—it’s here on earth that its greatest impact can be seen, from 5G to tackling climate change or ensuring we can all benefit through space inspired healthcare technologies such as these.”
- Adaptix, the company that developed the cutting-edge machine, was nurtured at ESA’s business incubation center in Harwell, UK.
- Nick Appleyard, head of Business Applications at the European Space Agency said: “This is a wonderful example of how ESA supports innovation. Adaptix started life in ESA’s UK Business Incubation Center and has grown to become a successful and innovative enterprise.”
- Mark Evans, chief executive of Adaptix Limited, said: “Working with ESA’s business incubation center hosted by the Rutherford Appleton Laboratory in Harwell has given us access to fantastic facilities and leading minds. ESA’s focus on commercializing space-heritage technology to create tangible benefits for the EU population and the UK economy has helped us to create 33 high-value UK jobs in research and development and, increasingly, in manufacturing.
- “Our vision is to create a business that will transform radiology through the export of high-science-content high-value products to achieve revenues of more than $100 m. X-ray is the primary diagnostic in healthcare and one day we hope that Adaptix technology will touch the life of everyone that you know.”
- The €1.2 m grant is due to come from an innovation fund drawn from ESA’s Business Applications and Space Solutions program, supported by the UK Space Agency.
• March 20, 2019: By surveying the center of our Galaxy, ESA’s XMM-Newton has discovered two colossal ‘chimneys’ funneling material from the vicinity of the Milky Way’s supermassive black hole into two huge cosmic bubbles. 60) 61)
- The giant bubbles were discovered in 2010 by NASA’s Fermi Gamma-ray Space Telescope: one stretches above the plane of the Milky Way galaxy and the other below, forming a shape akin to a colossal hourglass that spans about 50,000 light years – around half the diameter of the entire Galaxy. They can be thought of as giant ‘burps’ of material from the central regions of our Milky Way, where its central black hole, known as Sagittarius A*, resides.
- Now, XMM-Newton has discovered two channels of hot, X-ray emitting material streaming outwards from Sagittarius A*, finally linking the immediate surroundings of the black hole and the bubbles together.
- “We know that outflows and winds of material and energy emanating from a galaxy are crucial in sculpting and altering that galaxy’s shape over time – they are key players in how galaxies and other structures form and evolve throughout the cosmos,” says lead author Gabriele Ponti of the MPE (Max Planck Institute for Extraterrestrial Physics) in Garching, Germany, and the National Institute for Astrophysics in Italy.
- “Luckily, our Galaxy gives us a nearby laboratory to explore this in detail, and probe how material flows out into the space around us. We used data gathered by XMM-Newton between 2016 and 2018 to form the most extensive X-ray map ever made of the Milky Way’s core.”
- This map (Figure 60) revealed long channels of super-heated gas, each extending for hundreds of light years, streaming above and below the plane of the Milky Way.
- Scientists think that these act as a set of exhaust pipes through which energy and mass are transported from our Galaxy’s heart out to the base of the bubbles, replenishing them with new material.
- This finding clarifies how the activity occurring at the core of our home Galaxy, both present and past, is connected to the existence of larger structures around it.
- The outflow might be a remnant from our Galaxy’s past, from a period when activity was far more prevalent and powerful, or it may prove that even ‘quiescent’ galaxies – those that host a relatively quiet supermassive black hole and moderate levels of star formation like the Milky Way – can boast huge, energetic outflows of material.
- “The Milky Way is seen as a kind of prototype for a standard spiral galaxy,” says co-author Mark Morris of the University of California, Los Angeles, USA.
- “In a sense, this finding sheds light on how all typical spiral galaxies – and their contents – may behave across the cosmos.”


Legend to Figure 61: The two hot channels found by XMM-Newton stream outwards from Sagittarius A*, our Galaxy’s central supermassive black hole, and extend each for hundreds of light years, finally linking the immediate surroundings of the black hole and the bubbles together. Scientists think that these ‘chimneys’ act as a set of exhaust pipes through which energy and mass are transported from our Galaxy’s heart out to the base of the bubbles, replenishing them with new material.
- Despite its categorization as quiescent on the cosmic scale of galactic activity, previous data from XMM-Newton have revealed that our Galaxy’s core is still quite tumultuous and chaotic. Dying stars explode violently, throwing their material out into space; binary stars whirl around one another; and Sagittarius A*, a black hole as massive as four million Suns, lies in wait for incoming material to devour, later belching out radiation and energetic particles as it does so.
- Cosmic behemoths such as Sagittarius A* – and those even more massive – hosted by galaxies across the cosmos will be explored in depth by upcoming X-ray observatories like ESA’s Athena, the Advanced Telescope for High-Energy Astrophysics, scheduled for launch in 2031. Another future ESA mission, Lisa, the Laser Interferometer Space Antenna, will search for gravitational waves released by the merger of supermassive black holes at the core of distant, merging galaxies.
- Meanwhile, scientists are busy investigating these black holes with current missions like XMM-Newton.
- “There’s still a great deal to be done with XMM-Newton – the telescope could scan a significantly larger region of the Milky Way’s core, which would help us to map the bubbles and hot gas surrounding our Galaxy as well as their connections to the other components of the Milky Way, and hopefully figure out how all of this is linked together,” adds Gabriele.
- “Of course, we’re also looking forward to Athena and the breakthrough it will enable.”
- Athena will combine extremely high-resolution X-ray spectroscopy with excellent imaging capabilities over wide areas of the sky, allowing scientists to probe the nature and movement of hot cosmic gas like never before.
- “This outstanding result from XMM-Newton gives us an unprecedented view of what’s really happening at the core of the Milky Way, and presents the most extensive X-ray map ever created of the entire central region,” says ESA XMM-Newton Project Scientist Norbert Schartel.
- “This is especially exciting in the context of our upcoming missions. XMM-Newton is paving the way for the future generation of X-ray observatories, opening up abundant opportunities for these powerful spacecraft to make substantial new discoveries about our Universe.”
• February 25, 2019: Located some six million light-years away, the NGC 300 galaxy is relatively nearby. It is one of the closest galaxies beyond the Local Group – the hub of galaxies to which our own Milky Way galaxy belongs. Due to its proximity, it is a favorite target for astronomers to study stellar processes in spiral galaxies. 62)

- The population of stars in their prime is shown in this image in green hues, based on optical observations performed with the Wide Field Imager (WFI) on the MPG/ESO 2.2-meter telescope at La Silla, Chile. Red colors indicate the glow of cosmic dust in the interstellar medium that pervades the galaxy: this information derives from infrared observations made with NASA’s Spitzer space telescope, and can be used to trace stellar nurseries and future stellar generations across NGC 300.
- A complementary perspective on this galaxy’s composition comes from data collected in X-rays by ESA’s XMM-Newton space observatory, shown in blue. These represent the end points of the stellar life cycle, including massive stars on the verge of blasting out as supernovas, remnants of supernova explosions, neutron stars, and black holes. Many of these X-ray sources are located in NGC 300, while others – especially towards the edges of the image – are foreground objects in our own Galaxy, or background galaxies even farther away.
- The sizeable blue blob immediately to the left of the galaxy’s center is especially interesting, featuring two intriguing sources that are part of NGC 300 and shine brightly in X-rays.
- One of them, known as NGC 300 X-1, is in fact a binary system, consisting of a Wolf-Rayet star – an ageing hot, massive and luminous type star that drives strong winds into its surroundings – and a black hole, the compact remains of what was once another massive, hot star. As matter from the star flows towards the black hole, it is heated up to temperatures of millions of degrees or more, causing it to shine in X-rays.
- The other source, dubbed NGC 300 ULX1, was originally identified as a supernova explosion in 2010. However, later observations prompted astronomers to reconsider this interpretation, indicating that this source also conceals a binary system comprising a very massive star and a compact object – a neutron star or a black hole – feeding on material from its stellar companion.
- Data obtained in 2016 with ESA’s XMM-Newton and NASA’s NuSTAR observatories revealed regular variations in the X-ray signal of NGC 300 ULX1, suggesting that the compact object in this binary system is a highly magnetized, rapidly spinning neutron star, or pulsar.
- The large blue blob in the upper left corner is a much more distant object: a cluster of galaxies more than one billion light years away, whose X-ray glow is caused by the hot diffuse gas interspersed between the galaxies.
• January 28, 2019: Investigating the history of our cosmos with a large sample of distant ‘active’ galaxies observed by ESA’s XMM-Newton, a team of astronomers found there might be more to the early expansion of the Universe than predicted by the standard model of cosmology. 63) 64)
- According to the leading scenario, our Universe contains only a few percent of ordinary matter. One quarter of the cosmos is made of the elusive dark matter, which we can feel gravitationally but not observe, and the rest consists of the even more mysterious dark energy that is driving the current acceleration of the Universe’s expansion.
- This model is based on a multitude of data collected over the last couple of decades, from the cosmic microwave background, or CMB – the first light in the history of the cosmos, released only 380 000 years after the big bang and observed in unprecedented detail by ESA’s Planck mission – to more ‘local’ observations. The latter include supernova explosions, galaxy clusters and the gravitational distortion imprinted by dark matter on distant galaxies, and can be used to trace cosmic expansion in recent epochs of cosmic history – across the past nine billion years.
- A new study, led by Guido Risaliti of Università di Firenze, Italy, and Elisabeta Lusso of Durham University, UK, points to another type of cosmic tracer – quasars – that would fill part of the gap between these observations, measuring the expansion of the Universe up to 12 billion years ago.
- Quasars are the cores of galaxies where an active supermassive black hole is pulling in matter from its surroundings at very intense rates, shining brightly across the electromagnetic spectrum. As material falls onto the black hole, it forms a swirling disc that radiates in visible and ultraviolet light; this light, in turn, heats up nearby electrons, generating X-rays.
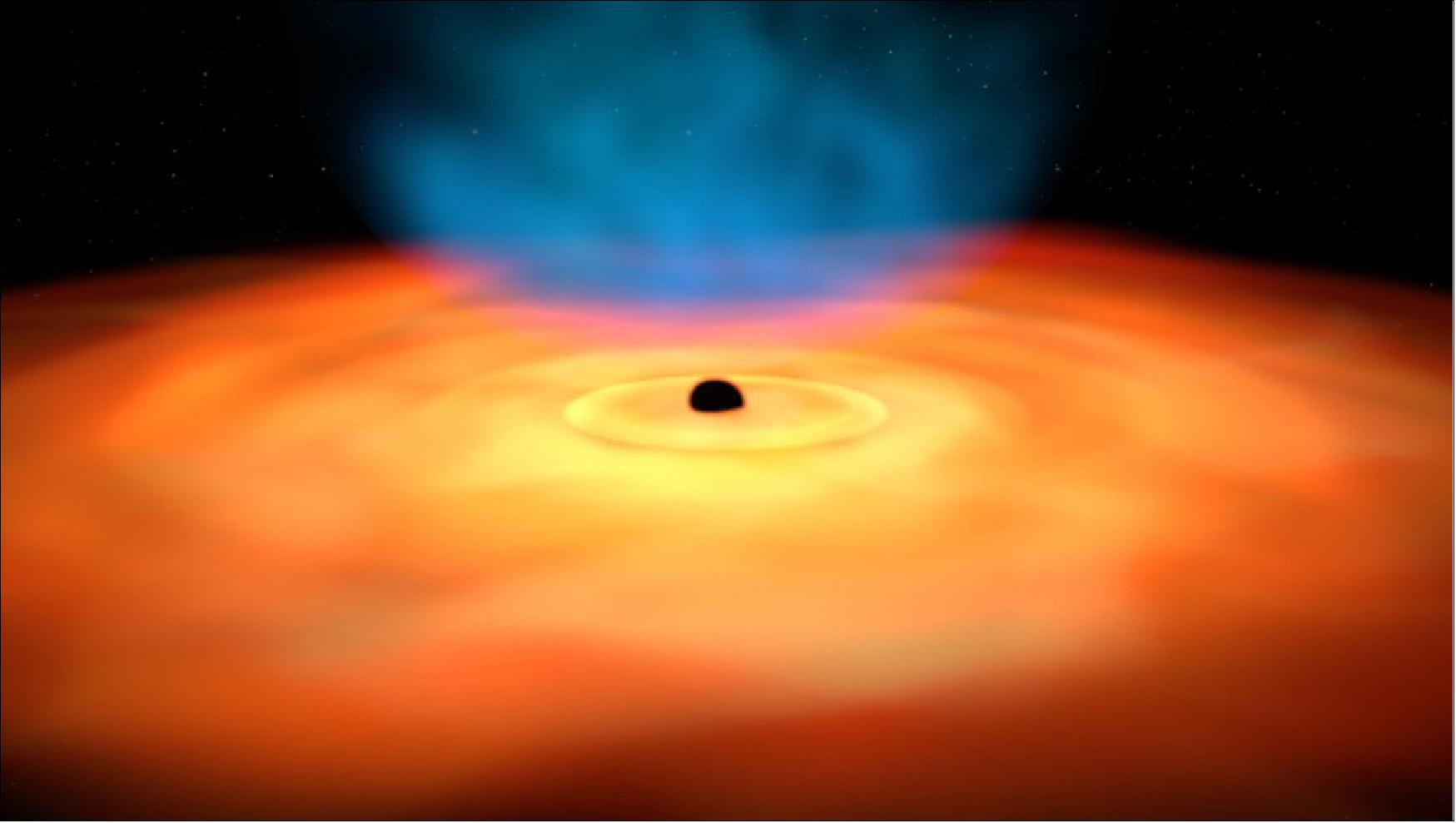
- Three years ago, Guido and Elisabeta realized that a well-known relation between the ultraviolet and X-ray brightness of quasars could be used to estimate the distance to these sources – something that is notoriously tricky in astronomy – and, ultimately, to probe the expansion history of the Universe.
- Astronomical sources whose properties allow us to gauge their distances are referred to as ‘standard candles’.
- The most notable class, known as ‘type-Ia’ supernova, consists of the spectacular demise of white dwarf stars after they have over-filled on material from a companion star, generating explosions of predictable brightness that allows astronomers to pinpoint the distance. Observations of these supernovas in the late 1990s revealed the Universe’s accelerated expansion over the last few billion years.
- “Using quasars as standard candles has great potential, since we can observe them out to much greater distances from us than type-Ia supernovas, and so use them to probe much earlier epochs in the history of the cosmos,” explains Elisabeta.
- With a sizeable sample of quasars at hand, the astronomers have now put their method into practice, and the results are intriguing.
- Digging into the XMM-Newton archive, they collected X-ray data for over 7000 quasars, combining them with ultraviolet observations from the ground-based Sloan Digital Sky Survey. They also used a new set of data, specially obtained with XMM-Newton in 2017 to look at very distant quasars, observing them as they were when the Universe was only about two billion years old. Finally, they complemented the data with a small number of even more distant quasars and with some relatively nearby ones, observed with NASA’s Chandra and Swift X-ray observatories, respectively.
- “Such a large sample enabled us to scrutinize the relation between X-ray and ultraviolet emission of quasars in painstaking detail, which greatly refined our technique to estimate their distance,” says Guido.
- The new XMM-Newton observations of distant quasars are so good that the team even identified two different groups: 70 percent of the sources shine brightly in low-energy X-rays, while the remaining 30 percent emit lower amounts of X-rays that are characterized by higher energies. For the further analysis, they only kept the earlier group of sources, in which the relation between X-ray and ultraviolet emission appears clearer.
- “It is quite remarkable that we can discern such level of detail in sources so distant from us that their light has been travelling for more than ten billion years before reaching us,” says Norbert Schartel, XMM-Newton project scientist at ESA.
- After skimming through the data and bringing the sample down to about 1600 quasars, the astronomers were left with the very best observations, leading to robust estimates of the distance to these sources that they could use to investigate the Universe’s expansion.
![Figure 64: The graph showing measurements of the distance to astronomical objects such as type-Ia supernovas (cyan symbols) and quasars (yellow, red and blue symbols) that can be used to study the expansion history of the Universe. Type-Ia supernovas are the most notable class of ‘standard candles’– astronomical sources whose properties allow us to gauge their distances. They consist of the spectacular demise of white dwarf stars after they have over-filled on material from a companion star, generating explosions of predictable brightness that allows astronomers to pinpoint the distance. Observations of these supernovas in the late 1990s revealed the Universe’s accelerated expansion over the last few billion years [image credit: Elisabeta Lusso & Guido Risaliti (2019)]](https://www.eoportal.org/ftp/satellite-missions/x/XMM-Newton_040722/XMM-Newton_Auto52.jpeg)
- “When we combine the quasar sample, which spans almost 12 billion years of cosmic history, with the more local sample of type-Ia supernovas, covering only the past eight billion years or so, we find similar results in the overlapping epochs,” says Elisabeta. “However, in the earlier phases that we can only probe with quasars, we find a discrepancy between the observed evolution of the Universe and what we would predict based on the standard cosmological model.”
- Looking into this previously poorly explored period of cosmic history with the help of quasars, the astronomers have revealed a possible tension in the standard model of cosmology, which might require the addition of extra parameters to reconcile the data with theory.
- “One of the possible solutions would be to invoke an evolving dark energy, with a density that increases as time goes by,” says Guido.
- Incidentally, this particular model would also alleviate another tension that has kept cosmologists busy lately, concerning the Hubble constant – the current rate of cosmic expansion. This discrepancy was found between estimates of the Hubble constant in the local Universe, based on supernova data – and, independently, on galaxy clusters – and those based on Planck’s observations of the cosmic microwave background in the early Universe.
- “This model is quite interesting because it might solve two puzzles at once, but the jury is definitely not out yet and we’ll have to look at many more models in great detail before we can solve this cosmic conundrum,” adds Guido.
- The team is looking forward to observing even more quasars in the future to further refine their results. Additional clues will also come from ESA’s Euclid mission, scheduled for a 2022 launch to explore the past ten billion years of cosmic expansion and investigate the nature of dark energy.
- “These are interesting times to investigate the history of our Universe, and it’s exciting that XMM-Newton can contribute by looking at a cosmic epoch that had remained largely unexplored so far,” concludes Norbert.
• January 9, 2019: Astronomers using ESA's XMM-Newton space observatory have studied a black hole devouring a star and discovered an exceptionally bright and stable signal that allowed them to determine the black hole’s spin rate. 65)
- Black holes are thought to lurk at the center of all massive galaxies throughout the Universe, and are inextricably tied to the properties of their host galaxies. As such, revealing more about these behemoths may hold the key to understanding how galaxies evolve over time.
- A black hole’s gravity is extreme, and can rip apart stars that stray too close. The debris from such torn-apart stars spirals inwards towards the hole, heats up, and emits intense X-rays.

- Despite the number of black holes thought to exist in the cosmos, many are dormant – there is no in-falling material to emit detectable radiation – and thus difficult to study. However, every few hundred thousand years or so, a star is predicted to pass near enough to a given black hole that it is torn apart. This offers a brief window of opportunity to measure some fundamental properties of the hole itself, such as its mass and the rate at which it is spinning.
- “It’s very difficult to constrain the spin of a black hole, as spin effects only emerge very close to the hole itself, where gravity is intensely strong and it’s difficult to see clearly,” says Dheeraj Pasham of the MIT Kavli Institute for Astrophysics and Space Research in Massachusetts, USA, and lead author of the new study. 66)
- “However, models show that the mass from a shredded star settles into a kind of inner disc that throws off X-rays. We guessed that finding instances where this disc glows especially brightly would be a good way to constrain a black hole's spin, but observations of such events weren’t sensitive enough to explore this region of strong gravity in detail – until now.”

- Dheeraj and colleagues studied an event called ASASSN-14li. ASASSN-14li was discovered by the ground-based All-Sky Automated Survey for SuperNovae (ASASSN) on 22 November 2014. The black hole tied to the event is at least one million times as massive as the Sun. “ASASSN-14li is nicknamed the ‘Rosetta Stone’ of these events,” adds Dheeraj. “All of its properties are characteristic of this type of event, and it has been studied by all currently operational major X-ray telescopes.”
- Using observations of ASASSN-14li from ESA’s XMM-Newton and NASA’s Chandra and Swift X-ray observatories, the scientists hunted for a signal that was both stable and showed a characteristic wave pattern often triggered when a black hole receives a sudden influx of mass – such as when devouring a passing star.
- “It’s an exceptional finding: such a bright signal that is stable for so long has never been seen before in the vicinity of any black hole,” adds co-author Alessia Franchini of the University of Milan, Italy. - “What’s more, the signal is coming from right near the black hole’s event horizon – beyond this point we can’t observe a thing, as gravity is so strong that even light can’t escape.”

- The study demonstrates a novel way to measure the spins of massive black holes: by observing their activity when they disrupt passing stars with their gravity. Such events may also help us to understand aspects of general relativity theory; while this has been explored extensively in ‘normal’ gravity, it is not yet fully understood in regions where gravity is exceptionally strong.
- “XMM-Newton is incredibly sensitive to these signals, more so than any other X-ray telescope,” says ESA’s XMM-Newton Project Scientist Norbert Schartel. “The satellite provides the long, uninterrupted, detailed exposures that are crucial to detecting signals such as these. “We’re only just beginning to understand the complex physics at play here. By finding instances where the mass from a shredded star glows especially brightly we can build a census of the black holes in the Universe, and probe how matter behaves in some of the most extreme areas and conditions in the cosmos.”
• November 21, 2018: Based on a new theoretical model, a team of scientists explored the rich data archive of ESA's XMM-Newton and NASA's Chandra space observatories to find pulsating X-ray emission from three sources. The discovery, relying on previous gamma-ray observations of the pulsars, provides a novel tool to investigate the mysterious mechanisms of pulsar emission, which will be important to understand these fascinating objects and use them for space navigation in the future. 67)
- Lighthouses of the Universe, pulsars are fast-rotating neutron stars that emit beams of radiation. As pulsars rotate and the beams alternatively point towards and away from Earth, the source oscillates between brighter and dimmer states, resulting in a signal that appears to 'pulse' every few milliseconds to seconds, with a regularity rivalling even atomic clocks.
- Pulsars are the incredibly dense, extremely magnetic, relics of massive stars, and are amongst the most extreme objects in the Universe. Understanding how particles behave in such a strong magnetic field is fundamental to understanding how matter and magnetic fields interact more generally.
- Originally detected through their radio emission, pulsars are now known to also emit other types of radiation, though typically in smaller amounts. Some of this emission is standard thermal radiation – the type that everything with a temperature above absolute zero emits. Pulsars release thermal radiation when they accrete matter, for example from another star.
- But pulsars also emit non-thermal radiation, as is often produced in the most extreme cosmic environments. In pulsars, non-thermal radiation can be created via two processes: synchrotron emission and curvature emission. Both processes involve charged particles being accelerated along magnetic field lines, causing them to radiate light that can vary in wavelength from radio waves to gamma-rays.
![Figure 68: XMM-Newton's view of pulsar J1826-1256 [image credit: ESA/XMM-Newton/J. Li, DESY (Deutsches Elektronen Synchrotron), Germany]](https://www.eoportal.org/ftp/satellite-missions/x/XMM-Newton_040722/XMM__Pulsar.gif)
- Non-thermal X-rays result mostly from synchrotron emission, while gamma-rays may come from so-called synchro-curvature emission – a combination of the two mechanisms. It is relatively easy to find pulsars that radiate gamma-rays – NASA's Fermi Gamma-Ray Space Telescope has detected more than 200 of them over the past decade, thanks to its ability to scan the whole sky. But only around 20 have been found to pulse in non-thermal X-rays.
- "Unlike gamma-ray detecting survey instruments, X-ray telescopes must be told exactly where to point, so we need to provide them with some sort of guidance," says Diego Torres, from the Institute of Space Sciences in Barcelona, Spain.
- Aware that there should be many pulsars emitting previously undetected non-thermal X-rays, Torres developed a model that combined synchrotron and curvature radiation to predict whether pulsars detected in gamma-rays could also be expected to appear in X-rays.
- "Scientific models describe phenomena that can't be experienced directly," explains Torres. -"This model in particular helps explain the emission processes in pulsars and can be used to predict the X-ray emission that we should observe, based on the known gamma-ray emission."
- The model describes the gamma-ray emission of pulsars detected by Fermi – specifically, the brightness observed at different wavelengths – and combines this information with three parameters that determine the pulsar emission. This allows a prediction of their brightness at other wavelengths, for instance in X-rays.
- Torres partnered with a team of scientists, led by Jian Li from the Deutsches Elektronen Synchrotron in Zeuthen near Berlin, Germany, to select three known gamma-ray emitting pulsars that they expected, based on the model, to also shine brightly in X-rays. They dug into the data archives of ESA's XMM-Newton and NASA's Chandra X-ray observatories to search for evidence of non-thermal X-ray emission from each of them.
- "Not only did we detect X-ray pulsations from all three of the pulsars, but we also found that the spectrum of X-rays was almost the same as predicted by the model," explains Li. — "This means that the model very accurately describes the emission processes within a pulsar."
- In particular, XMM-Newton data showed clear X-ray emission from PSR J1826-1256 – a radio quiet gamma-ray pulsar with a period of 110.2 milliseconds. The spectrum of light received from this pulsar was very close to that predicted by the model. X-ray emission from the other two pulsars, which both rotate slightly more quickly, was revealed using Chandra data.
- This discovery already represents a significant increase in the total number of pulsars known to emit non-thermal X-rays. The team expects that many more will be discovered over the next few years as the model can be used to work out where exactly to look for them.
- Finding more X-ray pulsars is important for revealing their global properties, including population characteristics. A better understanding of pulsars is also essential for potentially taking advantage of their accurate timing signals for future space navigation endeavors.

- The result is a step towards understanding the relationships between the emission by pulsars in different parts of the electromagnetic spectrum, enabling a robust way to predict the brightness of a pulsar at any given wavelength. This will help us better comprehend the interaction between particles and magnetic fields in pulsars and beyond.
- "This model can make accurate predictions of pulsar X-ray emission, and it can also predict the emission at other wavelengths, for example visible and ultraviolet," Torres continues. - "In the future, we hope to find new pulsars leading to a better understanding of their global properties."
- The study highlights the benefits of XMM-Newton's vast data archive to make new discoveries and showcases the impressive abilities of the mission to detect relatively dim sources. The team is also looking forward to using the next generation of X-ray space telescopes, including ESA's future Athena mission, to find even more pulsars emitting non-thermal X-rays. 68)
- "As the flagship of European X-ray astronomy, XMM-Newton is detecting more X-ray sources than any previous satellite. It is amazing to see that it is helping to solve so many cosmic mysteries," concludes Norbert Schartel, XMM-Newton Project Scientist at ESA.
Extended life for ESA's science missions 69)
• November 14, 2018: The SPC (Science Program Committee) of ESA has confirmed the continued operations of ten scientific missions in the Agency's fleet up to 2022. After a comprehensive review of their scientific merits and technical status, the SPC has decided to extend the operation of the five missions led by ESA's Science Program: Cluster, Gaia, INTEGRAL, Mars Express, and XMM-Newton. The SPC also confirmed the Agency's contributions to the extended operations of Hinode, Hubble, IRIS, SOHO, and ExoMars TGO. 69)
- This includes the confirmation of operations for the 2019–2020 cycle for missions that had been given indicative extensions as part of the previous extension process, and indicative extensions for an additional two years, up to 2022.
Note: Every two years, all missions whose approved operations end within the following four years are subject to review by the advisory structure of the Science Directorate. Extensions are granted to missions that satisfy the established criteria for operational status and science return, subject to the level of financial resources available in the science program. These extensions are valid for the following four years, subject to a mid-term review and confirmation after two years.
- The decision was taken during the SPC meeting at ESA/ESAC (European Space Astronomy Center) near Madrid, Spain, on 14 November.
- ESA's science missions have unique capabilities and are prolific in their scientific output. Cluster, for example, is the only mission that, by varying the separation between its four spacecraft, allows multipoint measurements of the magnetosphere in different regions and at different scales, while Gaia is performing the most precise astrometric survey ever realized, enabling unprecedented studies of the distribution and motions of stars in the Milky Way and beyond.
- Many of the science missions are proving to be of great value to pursue investigations that were not foreseen at the time of their launch. Examples include the role of INTEGRAL and XMM-Newton in the follow-up of recent gravitational wave detections, paving the way for the future of multi-messenger astronomy, and the many discoveries of diverse exoplanets by Hubble.
- Collaboration between missions, including those led by partner agencies, is also of great importance. The interplay between solar missions like Hinode, IRIS and SOHO provides an extensive suite of complementary instruments to study our Sun; meanwhile, Mars Express and ExoMars TGO are at the forefront of the international fleet investigating the Red Planet.
- Another compelling factor to support the extension is the introduction of new modes of operation to accommodate the evolving needs of the scientific community, as well as new opportunities for scientists to get involved with the missions.
• October 29, 2018: A gigantic cold front in the Perseus galaxy cluster has been observed by a trio of X-ray telescopes. The ancient cold front can be seen at the left of the image, drifting away from the much inner, younger front closer to the center. Galactic cold fronts are nothing like the cold fronts we experience on Earth – instead they are caused by galaxy clusters colliding into one another. The gravitational pull of a larger cluster tugs a smaller cluster closer, resulting in gas in the core of the cluster being sloshed around like liquid in a glass. This creates a cold front in a spiral pattern moving outwards from the core and these sloshing cold fronts can provide a probe of the intercluster medium. 70) 71) 72)
- Cold fronts are the oldest coherent structures in cool core clusters and this one has been moving away from the center of the cluster for over five billion years – longer than our Solar System has been in existence. The long curving structure spans around two million light years and is travelling at around 50 km/s.
- The Perseus galaxy cluster contains thousands of galaxies and a supermassive black hole at the center. The black hole is responsible for creating a harsh environment of sound waves and turbulence that should erode a cold front over time, smoothing out the previously sharp edges and creating gradual changes in density and temperature. Instead, the high-resolution Chandra image showed a surprisingly sharp edge on the cold front, and a temperature map revealed that the upper left of the cold front is split in two.
- The sharpness of the cold front suggests it has been preserved by strong magnetic fields wrapped around it, essentially acting as a shield against the harsh environment. This magnetic "draping" prevents the cold front from diffusing and is what has allowed it to survive so well for over five billion years as it drifts away from the center of the cluster.
- Aurora Simionescu and collaborators originally discovered the Perseus cold front in 2012 using data from ROSAT (the ROentgen SATellite), ESA's XMM-Newton Observatory, and Japan's Suzaku X-ray satellite. Chandra’s high-resolution X-ray vision allowed this more detailed work on the cold front to be performed.

• October 8, 2018: Astronomers using ESA’s XMM-Newton space observatory have captured the X-ray glow (shown here in purple in Figure 71) emitted by the hot gas that pervades the galaxy cluster XLSSC006. 73)
- The cluster is home to a few hundreds of galaxies, large amounts of diffuse, X-ray bright gas, and even larger amounts of dark matter, with a total mass equivalent to some 500 trillion solar masses. Because of its distance from us, we are seeing this galaxy cluster as it was when the Universe was only about nine billion years old.
- The galaxies that belong to the cluster are concentrated towards the center, with two dominant members. Since galaxy clusters normally have only one major galaxy at their core, this suggests that XLSSC006 is undergoing a merger event.
- The X-ray data were obtained as part of the XXL Survey, XMM-Newton’s largest observational program to date, with follow-up observations performed by a number of other observatories around the world and in space. The latest XXL Survey release contains data for 365 galaxy clusters, tracing their large-scale distribution across cosmic history. These observations are helping astronomers refine our understanding of the Universe’s structure and evolution, and will serve as a reference for ESA’s future missions Euclid and Athena.
![Figure 71: Pictured in this view, where the X-ray data are combined with a three-color composite of optical and near-infrared data from the Canada-France-Hawaii Telescope, are a multitude of other galaxies. Some are closer to us than the cluster – like the spiral galaxy towards the top right – and some are farther away. The image also shows a handful of foreground stars belonging to our Milky Way galaxy, which stand out with their diffraction spikes (a common artefact of astronomical images), while the small purple dots sprinkled across the frame are point sources of X-rays, many of them beyond the Milky Way [image credit:ESA/XMM-Newton (X-rays); CFHT-LS (optical); XXL Survey]](https://www.eoportal.org/ftp/satellite-missions/x/XMM-Newton_040722/XMM-Newton_Auto4C.jpeg)
• October 4, 2018: This mosaic shows the 365 galaxy clusters of the XXL Survey as imaged in X-rays by ESA's XMM-Newton space observatory. 74)
- The clusters are ordered by increasing distance from us, starting from the most nearby, at a redshift of 0.03, in the top left corner, all the way to the most distant one, at a redshift of 1.99 (the seventeenth cluster in the bottom row from the left); the last seven clusters in the bottom row have uncertain redshift.
- The XXL Survey is XMM-Newton's largest observational program to date. The second batch of data from the survey includes information on 365 galaxy clusters, which trace the large-scale structure of the Universe and its evolution through time, and on 26,000 active galactic nuclei (AGN).

• September 20, 2018: A UK team of astronomers report the first detection of matter falling into a black hole at 30% of the speed of light, located in the center of the billion-light year distant galaxy PG211+143. The team, led by Professor Ken Pounds of the University of Leicester, used data from the European Space Agency's X-ray observatory XMM-Newton to observe the black hole. Their results appear in a new paper in Monthly Notices of the Royal Astronomical Society. 75) 76)
- Black holes are objects with such strong gravitational fields that not even light travels quickly enough to escape their grasp, hence the description 'black'. They are hugely important in astronomy because they offer the most efficient way of extracting energy from matter. As a direct result, gas in-fall — accretion — onto black holes must be powering the most energetic phenomena in the Universe.
- The center of almost every galaxy — like our own Milky Way — contains a so-called supermassive black hole, with masses of millions to billions of times the mass of our Sun. With sufficient matter falling into the hole, these can become extremely luminous, and are seen as a quasar or AGN (Active Galactic Nucleus).
- However black holes are so compact that gas is almost always rotating too much to fall in directly. Instead it orbits the hole, approaching gradually through an accretion disk — a sequence of circular orbits of decreasing size. As gas spirals inwards, it moves faster and faster and becomes hot and luminous, turning gravitational energy into the radiation that astronomers observe.
- The orbit of the gas around the black hole is often assumed to be aligned with the rotation of the black hole, but there is no compelling reason for this to be the case. In fact, the reason we have summer and winter is that the Earth's daily rotation does not line up with its yearly orbit around the Sun.
- Until now it has been unclear how misaligned rotation might affect the in-fall of gas. This is particularly relevant to the feeding of supermassive black holes since matter (interstellar gas clouds or even isolated stars) can fall in from any direction.
- Using data from XMM-Newton, Prof. Pounds and his collaborators looked at X-ray spectra (where X-rays are dispersed by wavelength) from the galaxy PG211+143. This object lies more than one billion light years away in the direction of the constellation Coma Berenices, and is a Seyfert galaxy, characterized by a very bright AGN resulting from the presence of the massive black hole at its nucleus.
- The researchers found the spectra to be strongly red-shifted, showing the observed matter to be falling into the black hole at the enormous speed of 30% of the speed of light, or around 100,000 km/s. The gas has almost no rotation around the hole, and is detected extremely close to it in astronomical terms, at a distance of only 20 times the hole's size (its event horizon, the boundary of the region where escape is no longer possible).
- The observation agrees closely with recent theoretical work, also at Leicester and using the UK's Dirac supercomputer facility simulating the 'tearing' of misaligned accretion disks. This work has shown that rings of gas can break off and collide with each other, cancelling out their rotation and leaving gas to fall directly towards the black hole.
- Prof. Pounds, from the University of Leicester's Department of Physics and Astronomy, said: "The galaxy we were observing with XMM-Newton has a 40 million solar mass black hole which is very bright and evidently well fed. Indeed some 15 years ago we detected a powerful wind indicating the hole was being over-fed. While such winds are now found in many active galaxies, PG1211+143 has now yielded another 'first', with the detection of matter plunging directly into the hole itself."
- He continues: "We were able to follow an Earth-sized clump of matter for about a day, as it was pulled towards the black hole, accelerating to a third of the velocity of light before being swallowed up by the hole."
- A further implication of the new research is that 'chaotic accretion' from misaligned disks is likely to be common for supermassive black holes. Such black holes would then spin quite slowly, being able to accept far more gas and grow their masses more rapidly than generally believed, providing an explanation for why black holes which formed in the early Universe quickly gained very large masses.

• August 10, 2018: An enigmatic X-ray source revealed as part of a data-mining project for high-school students shows unexplored avenues hidden in the vast archive of ESA’s XMM-Newton X-ray Observatory. 77) 78)
![Figure 74: Flaring source in NGC 6540: A peculiar X-ray source spotted in the globular cluster NGC 6540 as part of a collaboration between scientists at the National Institute of Astrophysics (INAF) in Milan, Italy, and a group of students from a local high school. In 2005, ESA’s XMM-Newton saw this source undergo a flare that boosted the luminosity of the source by up to 50 times its normal level for about five minutes. Too short to be an ordinary stellar flare, but too faint to be linked to a compact object, this event is challenging our understanding of X-ray outbursts [image credit: ESA/XMM-Newton, A. De Carlo (INAF)] 79)](https://www.eoportal.org/ftp/satellite-missions/x/XMM-Newton_040722/XMM%20Flaring_source.gif)
- When XMM-Newton was launched in 1999, most students who are finishing high school today were not even born. Yet ESA's almost two-decade old X-ray observatory has many surprises to be explored by the next generation of scientists.
- A taste of new discoveries was unveiled in a recent collaboration between scientists at the National Institute of Astrophysics (INAF) in Milan, Italy, and a group of twelfth-grade students from a secondary school in nearby Saronno.
- The fruitful interaction was part of the Exploring the X-ray Transient and variable Sky project, EXTraS, an international research study of variable sources from the first 15 years of XMM-Newton observations.
- "We recently published the EXTraS catalog, which includes all the X-ray sources – about half a million – whose brightness changes over time as observed by XMM-Newton, and lists several observed parameters for each source," says Andrea De Luca, one of the scientists who coordinated the student project.
- "The next step was to delve into this vast data set and find potentially interesting sources, and we thought this would be an exciting challenge for a student internship," adds Andrea.
- Scientists at INAF in Milan have been cooperating with local schools for a few years, hosting several groups of students at the institute for a couple of weeks and embedding them in the activities of the various research groups.
- "For this particular project, the students received an introduction about astronomy and the exotic sources we study with X-ray telescopes, as well as a tutorial on the database and how to use it," explains Ruben Salvaterra, another scientist involved in the program. "Once they were ready to explore the data archive, they proved very effective and resourceful."
- The six students analyzed about 200 X-ray sources, looking at their light curve – a graph showing the object's variability over time – and checking the scientific literature to verify whether they had been studied already.
- Eventually, they identified a handful of sources exhibiting interesting properties – a powerful flare, for example – that had not been previously reported by other studies. "One of the sources stood out as especially intriguing," says Andrea.
- Featuring the shortest flare of all analyzed objects, this source appears to be located in the globular cluster NGC 6540 – a dense grouping of stars – and had not been studied before.
- After presenting their findings to the scientists in a seminar, the students went back to school. But the work for Andrea, Ruben and collaborators had only just begun.
- "The source identified by the students displays brightness changes like no other known objects, so we started looking more in detail," says Ruben.
- An otherwise low-luminosity source of X-rays, XMM-Newton saw it brighten by up to 50 times its normal level in 2005, and quickly fall again after about five minutes.
- Stars like our Sun shine moderately in X-rays, and occasionally undergo flares that boost their brightness like the one observed in this source. However, such events normally last much longer – up to a few hours or even days.
- On the other hand, short outbursts are observed in binary star systems hosting a dense stellar remnant such as neutron star, but these outpourings of X-rays are characterized by a much higher luminosity.
- "This event is challenging our understanding of X-ray outbursts: too short to be an ordinary stellar flare, but too faint to be linked to a compact object," explains collaborator Sandro Mereghetti, lead author of the paper presenting the results.

- Another possibility is that the source is a so-called chromospherically active binary, a dual system of stars with intense X-ray activity caused by processes in their chromosphere, an intermediate layer in a star's atmosphere. But even in this case, it does not closely match the properties of any known object of this class.
- The scientists suspect that this peculiar source is not unique, and that other objects with similar properties are lurking in the XMM-Newton archive but have not yet been identified because of the combination of low luminosity and short duration of the flare.
- "The systematic study of variability that led to the compilation of the EXTraS catalog, together with this first attempt at data mining, suggests that we have opened a new, unexplored window on the X-ray Universe," adds Sandro. - The team plans to study the newly identified source in greater detail to better understand its nature, while searching for more similar objects in the archive.
- "It is exciting to find hidden jewels like this source in the XMM-Newton archive, and that young students are helping us find them while learning and having fun," concludes Norbert Schartel, XMM-Newton project scientist at ESA.
• July 25, 2018: Members of the X-ray astronomy working group at the Leibniz Institute for Astrophysics (AIP) in Potsdam and an international team have published the first catalog of X-ray sources in multiple observed sky regions. The catalog comprises almost 72,000 objects, partly of exotic nature, which were observed with the space-based X-ray telescope XMM-Newton. It provides information on the physical properties of the sources and enables astronomers to identify brightness variations on time scales of several years - and includes several thousand new detections. 80) 81)
- Since its launch end of 1999, the European X-ray satellite XMM-Newton has observed many patches of the sky repeatedly. Members of the X-ray astronomy group have developed new software to search for astrophysical objects in overlapping observations and used it to compile the first catalog. By combining multiple observations of the same region of sky, higher accuracy is reached and faint sources are found that are not detectable in the individual observations. "Our method is similar to combining several transparencies showing the same subject: The more images are superimposed the more details become visible," explains Dr. Iris Traulsen, the project scientist at the AIP.

- The new catalog comprises 71,951 X-ray sources in 1,789 XMM-Newton observations and lists a wealth of information on their physical properties. Several thousand of these sources are newly discovered, many of them very faint and difficult to detect. The catalog can be used to trace brightness changes of X-ray sources over time scales of up to 14.5 years. Dr. Axel Schwope, team leader at the AIP, says: "Variations of the X-ray brightness are an essential criteria used to search for exotic Celestial objects. To decipher their nature, we also employ the LBT (Large Binocular Telescope) in Arizona." The AIP is one of the LBT partners and contributes to its instrumentation and software.
- Scientists all over the world have been using the XMM-Newton Source Catalogs to get new information about their research objects and to search for rare and as yet unknown sources of X-rays. 82)
• June 20, 2018: After a nearly twenty-year long game of cosmic hide-and-seek, astronomers using ESA's XMM-Newton space observatory have finally found evidence of hot, diffuse gas permeating the cosmos, closing a puzzling gap in the overall budget of 'normal' matter in the Universe. 83)

- While the mysterious dark matter and dark energy make up about 25 and 70 percent of our cosmos respectively, the ordinary matter that makes up everything we see – from stars and galaxies to planets and people – amounts to only about five percent. - But even this five percent turns out to be quite hard to track down.
- The total amount of ordinary matter, which astronomers refer to as baryons, can be estimated from observations of the Cosmic Microwave Background, which is the most ancient light in the history of the Universe, dating back to only about 380 000 years after the Big Bang.
- Observations of very distant galaxies allow astronomers to follow the evolution of this matter throughout the Universe's first couple billions of years. After that, however, more than half of it seemed to have gone missing.
- "The missing baryons represent one of the biggest mysteries in modern astrophysics," explains Fabrizio Nicastro, lead author of the paper presenting a solution to the mystery, published today in Nature. "We know this matter must be out there, we see it in the early Universe, but then we can no longer get hold of it. Where did it go?" 84)
- Counting the population of stars in galaxies across the Universe, plus the interstellar gas that permeates galaxies – the raw material to create stars – only gets as far as a mere ten percent of all ordinary matter. Adding up the hot, diffuse gas in the haloes that encompass galaxies and the even hotter gas that fills galaxy clusters, which are the largest cosmic structures held together by gravity, raises the inventory to less than twenty percent.
- This is not surprising: stars, galaxies and galaxy clusters form in the densest knots of the cosmic web, the filamentary distribution of both dark and ordinary matter that extends throughout the Universe. While these sites are dense, they are also rare, so not the best spots to look for the majority of cosmic matter.
- Astronomers suspected that the 'missing' baryons must be lurking in the ubiquitous filaments of this cosmic web, where matter is however less dense and therefore more challenging to observe. Using different techniques over the years, they were able to locate a good chunk of this intergalactic material – mainly its cool and warm components – bringing up the total budget to a respectable 60 percent, but leaving the overall mystery still unsolved.

- Fabrizio and many other astronomers around the world have been on the tracks of the remaining baryons for almost two decades, ever since X-ray observatories such as ESA's XMM-Newton and NASA's Chandra became available to the scientific community.
- Observing in this portion of the electromagnetic spectrum, they can detect hot intergalactic gas, with temperatures around a million degrees or more, that is blocking the X-rays emitted by even more distant sources.
- For this project, Fabrizio and his collaborators used XMM-Newton to look at a quasar – a massive galaxy with a supermassive black hole at its center that is actively devouring matter and shining brightly from X-rays to radio waves. They observed this quasar, whose light takes more than four billion years to reach us, for a total of 18 days, split between 2015 and 2017, in the longest X-ray observation ever performed of such a source.
- "After combing through the data, we succeeded at finding the signature of oxygen in the hot intergalactic gas between us and the distant quasar, at two different locations along the line of sight," says Fabrizio. "This is happening because there are huge reservoirs of material – including oxygen – lying there, and just in the amount we were expecting, so we finally can close the gap in the baryon budget of the Universe."
- This extraordinary result is the beginning of a new quest. Observations of different sources across the sky are needed to confirm whether these findings are truly universal, and to further investigate the physical state of this long-sought-for matter.
- Fabrizio and his colleagues are planning to study more quasars with XMM-Newton and Chandra in the coming years. To fully explore the distribution and properties of this so-called warm-hot intergalactic medium, however, more sensitive instruments will be needed, like ESA's Athena, the Advanced Telescope for High-Energy Astrophysics, scheduled for launch in 2028.
- "The discovery of the missing baryons with XMM-Newton is the exciting first step to fully characterize the circumstances and structures in which these baryons are found," says co-author Jelle Kaastra from the Netherlands Institute for Space Research. "For the next steps, we will need the much higher sensitivity of Athena, which has the study of the warm-hot intergalactic medium as one of its main goals, to improve our understanding of how structures grow in the history of the Universe."
- "It makes us very proud that XMM-Newton was able to discover the weak signal of this long elusive material, hidden in a million-degree hot fog that extends through intergalactic space for hundreds of thousands of light years," says Norbert Schartel, XMM-Newton project scientist at ESA. "Now that we know these baryons are no longer missing, we can't wait to study them in greater detail."
• June 18, 2018: ESA’s XMM-Newton observatory has discovered the best-ever candidate for a very rare and elusive type of cosmic phenomenon: a medium-weight black hole in the process of tearing apart and feasting on a nearby star. 85) 86)
- There are various types of black hole lurking throughout the Universe: massive stars create stellar-mass black holes when they die, while galaxies host supermassive black holes at their centers, with masses equivalent to millions or billions of Suns.
- Lying between these extremes is a more retiring member of the black hole family: intermediate-mass black holes. Thought to be seeds that will eventually grow to become supermassive, these black holes are especially elusive, and thus very few robust candidates have ever been found.
- Now, a team of researchers using data from ESA’s XMM-Newton X-ray space observatory, as well as NASA’s Chandra X-Ray Observatory and Swift X-Ray Telescope, has found a rare telltale sign of activity. They detected an enormous flare of radiation in the outskirts of a distant galaxy, thrown off as a star passed too close to a black hole and was subsequently devoured.
- “This is incredibly exciting: this type of black hole hasn’t been spotted so clearly before,” says lead scientist Dacheng Lin of the University of New Hampshire, USA. “A few candidates have been found, but on the whole they’re extremely rare and very sought after. This is the best intermediate-mass black hole candidate observed so far.”
- This breed of black hole is thought to form in various ways. One formation scenario is the runaway merger of massive stars lying within dense star clusters, making the centers of these clusters one of the best places to hunt for them. However, by the time such black holes have formed, these sites tend to be devoid of gas, leaving the black holes with no material to consume and thus little radiation to emit – which in turn makes them extremely difficult to spot.
- "One of the few methods we can use to try to find an intermediate-mass black hole is to wait for a star to pass close to it and become disrupted – this essentially 'activates' the black hole's appetite again and prompts it to emit a flare that we can observe," adds Lin. "This kind of event has only been clearly seen at the center of a galaxy before, not at the outer edges."
- Lin and colleagues sifted through data from XMM-Newton to find the candidate. They identified it in observations of a large galaxy some 740 million light-years away, taken in 2006 and 2009 as part of a galaxy survey, and in additional data from Chandra (2006 and 2016) and Swift (2014).
- "We also looked at images of the galaxy taken by a whole host of other telescopes, to see what the emission looked like optically," says co-author Jay Strader of Michigan State University, USA. "We spotted the source flaring in brightness in two images from 2005 – it appeared far bluer and brighter than it had just a few years previously. By comparing all the data we determined that the unfortunate star was likely disrupted in October 2003 in our time, and produced a burst of energy that decayed over the following 10 years or so."
- The scientists believe that the star was disrupted and torn apart by a black hole with a mass of around fifty thousand times that of the Sun.
- Such star-triggered outbursts are expected to only happen rarely from this type of black hole, so this discovery suggests that there could be many more lurking in a dormant state in galaxy peripheries across the local Universe.
- "This candidate was discovered via an intensive search of XMM-Newton's X-ray Source Catalog, which is filled with high-quality data covering large areas of sky, essential for determining how large the black hole was and what happened to cause the observed burst of radiation," says Norbert Schartel, ESA Project Scientist for XMM-Newton.
- "The XMM-Newton X-ray Source Catalogue is presently the largest catalogue of this type, containing more than half a million sources: exotic objects like the one discovered in our study are still hidden there and waiting to be discovered through intensive data mining," adds co-author Natalie Webb, director of the XMM-Newton Survey Science Center at the Research Institute in Astrophysics and Planetology (IRAP) in Toulouse, France.
- "Learning more about these objects and associated phenomena is key to our understanding of black holes. Our models are currently akin to a scenario in which an alien civilization observes Earth and spots grandparents dropping their grandchildren at pre-school: they might assume that there's something intermediate to fit their model of a human lifespan, but without observing that link, there's no way to know for sure. This finding is incredibly important, and shows that the discovery method employed here is a good one to use," concludes Norbert.


• June 11, 2018: This turbulent celestial palette of purple and yellow shows a bubble of gas named NGC 3199 , blown by a star known as WR18 (Wolf-Rayet 18). 87)
- Wolf-Rayet stars are massive, powerful, and energetic stars that are just about reaching the end of their lives. They flood their surroundings with thick, intense, fast-moving winds that push and sweep at the material found there, carving out weird and wonderful shapes as they do so. These winds can create strong shockwaves when they collide with the comparatively cool interstellar medium, causing them to heat up anything in their vicinity. This process can heat material to such high temperatures that it is capable of emitting X-rays, a type of radiation emitted only by highly energetic phenomena in the Universe.
- This is what has happened in the case of NGC 3199. Although this kind of scenario has been seen before, it is still relatively rare; only three other Wolf-Rayet bubbles have been seen to emit X-rays (NGC 2359, NGC 6888, and S308). WR18 is thought to be a star with especially powerful winds; once it has run out of material to fuel these substantial winds it will explode violently as a supernova, creating a final breath-taking blast as it ends its stellar life.
- The image of Figure 81 was taken by the EPIC (European Photon Imaging Camera) on ESA’s XMM-Newton X-ray space observatory, and marks different patches of gas in different colors. The incredibly hot, diffuse, X-ray-emitting gas within the Wolf-Rayet bubble is shown in blue, while a bright arc that is visible in the optical part of the spectrum is traced out in shades of yellow-green (oxygen emission) and red (sulphur emission).
- The blue and yellow-green component forms an optical nebula – a glowing cloud of dust and ionized gases – that stretches out towards the western end of the X-ray bubble (in this image, North is to the upper left). This lopsided arc caused astronomers to previously identify WR18 as a so-called runaway star moving far faster than expected in relation to its surroundings, but more recent studies have shown that the observed X-ray emission does not support this idea. Instead, the shape of NGC 3199 is thought to be due to variations in the chemistry of the bubble’s surroundings, and the initial configuration of the interstellar medium around WR18.

• May 31, 2018: Last year, the first detection of gravitational waves linked to a gamma-ray burst triggered a vast follow-up campaign with ground and space telescopes to study the aftermath of the neutron star merger that gave rise to the explosion. ESA's XMM-Newton observations, obtained a few months after the discovery, caught the moment when its X-ray emission stopped increasing, opening new questions about the nature of this peculiar source. 88)

- Gravitational waves, predicted by Albert Einstein's general theory of relativity in 1918, are ripples in the fabric of spacetime caused by accelerating massive objects like colliding pairs of neutron stars or black holes.
- These fluctuations, which remained elusive for a century after the prediction, can now be detected using giant experiments on the ground such as the Laser Interferometer Gravitational-wave Observatory (LIGO) in the United States and Europe's Virgo interferometer.
- After a gravitational wave detection, scientists mobilize a large number of ground-based and spaceborne astronomical facilities to look for a possible counterpart of the waves across the electromagnetic spectrum and learn more about their source.
- All but one of the six gravitational-wave events that have been observed since 2015 had no evidence of an electromagnetic counterpart, in agreement with the fact that they originated from the merger of black holes – a cosmic phenomenon that is not expected to release any light.
- This is why the first detection of gravitational waves jointly with gamma rays, on 17 August 2017, gave rise to a worldwide sensation, launching an observing campaign that involved observatories across the globe and in space to follow the evolution of this never-before-seen phenomenon.
- ESA's INTEGRAL and NASA's Fermi gamma-ray satellites had detected the blast only two seconds after its gravitational waves had passed through the LIGO and Virgo detectors, linking the gamma-ray burst to the source of the spacetime ripples, caused by the coalescence of two neutron stars – dense remnants that form at the end of a massive star's life.
- Scientists then looked for the afterglow of the explosion created by the neutron star merger, which they expected to observe at longer wavelengths, from X-rays to radio waves. While the optical signal was received about half a day after the original detection, it took no less than nine days for the first observations of this object in X-rays and radio waves.
- The delay of the X-ray and radio afterglow contains information about the geometry of the explosion, suggesting that it might have generated two symmetric and collimated jets, neither of which, however, pointed towards Earth.
- The X-ray observations were performed with NASA's Chandra X-ray Observatory and other space telescopes. Chandra kept an eye on this source during the following months, recording an ever increasing trend in its X-ray brightness.
- Due to observational constraints, XMM-Newton could not observe the aftermath of this cosmic clash for the first four months after its first detection. When it eventually did so, on 29 December 2017, the X-ray brightness seemed to have stopped rising.
- "The XMM-Newton observations had a very good timing," explains Paolo D'Avanzo from INAF – Osservatorio Astronomico di Brera, Italy. D'Avanzo is the lead author of the paper reporting the results, published this month in Astronomy & Astrophysics. 89)
- "By measuring the same value seen by Chandra earlier that month, XMM-Newton provided the first evidence that the source had reached its X-ray peak, and that its incessant brightening had finally come to a halt," he adds. "This was later confirmed by another team of scientists who keep monitoring the source with Chandra."
- Scientists expected that the X-ray brightness would reach a peak after a few months, as the material that had been ejected and heated up by the explosion slowly decelerated into the surrounding interstellar medium. The further evolution of the system, however, could still have some surprises in store.
- If the explosion did produce two symmetric jets that are not pointing towards Earth, as inferred from the first observations, its X-ray output will decrease rapidly.
- But there is another possibility that could explain the data obtained so far: the explosion could have also happened as a spherical 'fireball', without jets, but with a much lower energy. In this case, the X-ray brightness would decrease at a more leisurely pace after the peak.
- "We are eager to see how this source will behave over the coming months, since it will tell us whether we are looking off-axis at a beamed gamma-ray burst, as we thought until now, or witnessing a different phenomenon," says D'Avanzo. "This coincidentally well-timed observation is taking us one step closer to understanding the nature of this unique source," says Norbert Schartel, XMM-Newton Project Scientist at ESA.
- In what scientists call a multi-messenger approach, observations across the electromagnetic spectrum are key to study in-depth this and similar sources of gravitational waves that will be discovered in future years by LIGO and Virgo.
- The two gravitational wave experiments will start their observations again, with improved sensitivity, at the beginning of 2019, while ESA's future mission, LISA, the Laser Interferometer Space Antenna, which will observe lower frequency gravitational waves from space, is planned for launch in 2034.

• April 18, 2018: Astronomers using ESA’s XMM-Newton space observatory have probed the gas-filled haloes around galaxies in a quest to find ‘missing’ matter thought to reside there, but have come up empty-handed – so where is it? 90)
- All the matter in the Universe exists in the form of ‘normal’ matter or the notoriously elusive and invisible dark matter, with the latter around six times more prolific.
- Curiously, scientists studying nearby galaxies in recent years have found them to contain three times less normal matter than expected, with our own Milky Way Galaxy containing less than half the expected amount. “This has long been a mystery, and scientists have spent a lot of effort searching for this missing matter,” says Jiangtao Li of the University of Michigan, USA, and lead author of a new paper. 91) “Why is it not in galaxies — or is it there, but we are just not seeing it? If it’s not there, where is it? It is important we solve this puzzle, as it is one of the most uncertain parts of our models of both the early Universe and of how galaxies form.”
![Figure 84: This image illustrates the X-ray emission around a set of five galaxies that have been stacked together to bring out the details in their spherical, gaseous haloes. It was created by a team of scientists using ESA’s XMM-Newton space observatory, with the X-ray emission highlighted in purple [image credit: ESA/XMM-Newton; J-T. Li (University of Michigan, USA); Sloan Digital Sky Survey (SDSS)] 92)](https://www.eoportal.org/ftp/satellite-missions/x/XMM-Newton_040722/XMM-Newton_Auto41.jpeg)
- Rather than lying within the main bulk of the galaxy, the part can be observed optically, researchers thought it may instead lie within a region of hot gas that stretches further out into space to form a galaxy’s halo.
- These hot, spherical haloes have been detected before, but the region is so faint that it is difficult to observe in detail – its X-ray emission can become lost and indistinguishable from background radiation. Often, scientists observe a small distance into this region and extrapolate their findings but this can result in unclear and varying results.
- Jiangtao and colleagues wanted to measure the hot gas out to larger distances using ESA’s XMM-Newton X-ray space observatory. They looked at six similar spiral galaxies and combined the data to create one galaxy with their average properties.
- “By doing this, the galaxy’s signal becomes stronger and the X-ray background becomes better behaved,” adds co-author Joel Bregman, also of the University of Michigan. “We were then able to see the X-ray emission to about three times further out than if observing a single galaxy, which made our extrapolation more accurate and reliable.”
- Massive and isolated spiral galaxies offer the best chance to search for missing matter. They are massive enough to heat gas to temperatures of millions of degrees so that they emit X-rays, and have largely avoided being contaminated by other material through star formation or interactions with other galaxies.
Still Missing
- The team’s results showed that the halo surrounding galaxies like the ones observed cannot contain all of the missing matter after all. Despite extrapolating out to almost 30 times the radius of the Milky Way, nearly three-quarters of the expected material was still missing.
- There are two main alternative theories as to where it could be: either it is stored in another gas phase that is poorly observed – perhaps either a hotter and more tenuous phase or a cooler and denser one – or within a patch of space that is not covered by our current observations or emits X-rays too faintly to be detected.
- Either way, since the galaxies do not contain enough missing matter they may have ejected it out into space, perhaps driven by injections of energy from exploding stars or by supermassive black holes.
- “This work is important to help create more realistic galaxy models, and in turn help us better understand how our own Galaxy formed and evolved,” says Norbert Schartel, ESA XMM-Newton project scientist. “This kind of finding is simply not possible without the incredible sensitivity of XMM-Newton. - In the future, scientists can add even more galaxies to our study samples and use XMM-Newton in collaboration with other high-energy observatories, such as ESA’s upcoming ATHENA (Advanced Telescope for High-ENergy Astrophysics) mission, to probe the extended, low-density parts of a galaxy’s outer edges, as we continue to unravel the mystery of the Universe’s missing matter.”
• March 19, 2018: The Crab Nebula is a supernova remnant some 6500 light-years from Earth in the constellation of Taurus. At the center of the nebula is a pulsar – the remnant of a star that exploded to form the nebula. The pulsar rotates around 30 times a second, sweeping a beam of radio waves across the Galaxy. Some of the material surrounding the pulsar was ejected before the star exploded, and the rest was expelled during the supernova. The wind from the pulsar escapes at high speed, creating a dynamic structure by interacting with the ejected material. 93)
- The nebula is currently expanding at around 1500 km/s, as revealed by images taken a few years apart. By tracing this backwards it is possible to pinpoint the year in which the star exploded, and this coincides with observations by Chinese astronomers in 1054 of a star bright enough to be seen during daylight.
- The image shown in Figure 85 is in ultraviolet light taken by ESA’s XMM-Newton telescope, which has been surveying the sky since 2000. While this is primarily a telescope for observing X-rays, the Optical Monitor enables optical and ultraviolet observations to be made simultaneously with X-ray observations. The image is a composite of 75 individual images taken between 2001 and 2015. Very few ultraviolet images of the Crab Nebula were available before this one.
- The ultraviolet emission is thought to come from ‘synchrotron radiation’, created when atomic particles spiral around magnetic field lines. The XMM-Newton image reveals ‘bays’ indenting the east and west sides of the nebula. It is thought that a magnetized torus of material surrounded the star before it exploded, which then blocked the high-speed particles and thus the synchrotron radiation. The bays are also evident in radio images, although the eastern bay is better defined owing to intricate features around the borders of the radio image.
- A new composite of the Crab Nebula comprising NASA Chandra and Spitzer data and NASA/ESA Hubble data was also released last week.

• February 26, 2018: In 2014, ESA's XMM-Newton spotted X-rays emanating from the massive star Rho Ophiuchi A and, last year, found these to ebb and flow periodically in the form of intense flares – both unexpected results. The team has now used ESO's VLT (Very Large Telescope) to find that the star boasts a strong magnetic field, confirming its status as a cosmic lighthouse. 94)

- Stars like the Sun are known to produce strong X-ray flares, but massive stars appear to be very different. In stars upwards of eight solar masses X-ray emission is steady, and no such star had been confidently observed to repeatedly flare in this part of the spectrum – until recently.
In 2014, a team of scientists used ESA's XMM-Newton space observatory to observe a massive star named Rho Ophiuchi A. This star sits at the heart of the Rho Ophiuchi Dark Cloud, a nearby region known to be actively forming new stars. Surprisingly, the data showed an abundance of X-rays streaming out from the star, prompting the team to look closer.
"We observed the star with XMM-Newton for almost 40 hours and found something even more unexpected," says Ignazio Pillitteri of the INAF–Osservatorio Astronomico di Palermo, Italy, and leader of the research team.
"Rather than a smooth, steady emission, the X-rays pulsed periodically outwards from Rho Ophiuchi A, varying over a period of roughly 1.2 days as the star rotated – like an X-ray lighthouse! This is quite a new phenomenon in stars bigger than the Sun."

- Rho Ophiuchi A is far hotter and more massive than our parent star. It remains unknown how X-rays are generated in such stellar heavyweights; one possibility is a strong intrinsic magnetism, which would be observable via signs of surface magnetism. However, how such a magnetic field would come to be – and how it would be linked to any X-ray emission – remains unclear.
- "We guessed that there may be a giant active magnetic spot on the surface of Rho Ophiuchi A – a bit like a sunspot, only far bigger and more stable," adds Pillitteri. "As the star rotates, this spot would come in and out of view, causing the observed pulsing X-rays. However, this idea was somewhat unlikely; spots on stars form when an interior magnetic field pops out to the surface, and we know that only one in ten massive stars has a measurable magnetic field."
- Another way the pulsing 'lighthouse effect' could be created is via a lower-mass orbiting companion that added its own copious X-rays to the light attributed to Rho Ophiuchi A; this X-ray emission would vary in strength as the hypothetical smaller star crossed in front of and behind Rho Ophiuchi A during its 1.2-day orbit. The team also considered this possibility: that Rho Ophiuchi A could have a small, unseen, lower-mass companion in a very tight orbit.
- "To find out one way or another, we rushed to get magnetic measurements of Rho Ophiuchi A using one of the largest ground-based telescopes in existence: ESO's Very Large Telescope," says Lida Oskinova of the University of Potsdam, Germany, a member of the international team that conducted the study. "Excitingly, these measurements confirmed one of our predictions and showed that the X-rays are most likely linked to magnetic structures on the surface of the star."
- These measurements were made in visible light using a technique known as spectropolarimetry, which involves studying various wavelengths of polarized light emanating from a star. The data showed Rho Ophiuchi A to have an intense magnetic field some 500 times stronger than that of the Sun. 95) 96) 97)
- "Such a strong field is easily capable of producing the kind of flares we spotted," says Pillitteri. "This confirms that what we discovered using XMM-Newton were indeed X-ray flares on Rho Ophiuchi A, that massive stars can be magnetically active – as shown by the optical observations – and that this activity can be seen in X-rays."
- The combined data indicate that Rho Ophiuchi A is the only star of its type to have a confirmed active magnetic region on its surface that emits X-rays. Hunting for similar behavior in stars like Rho Ophiuchi A will help scientists to understand how prevalent this phenomenon is, and unravel more about the magnetic properties of such stars.
- "This study is an important one in our exploration of massive stars – there's much we still don't understand about these objects," says Norbert Schartel, ESA XMM-Newton Project Scientist. "Together, the extraordinary capabilities of XMM-Newton and the Very Large Telescope have now uncovered another piece of the puzzle."
- "As a bonus, it illustrates the process of science very well – of finding something interesting or unusual, investigating and coming up with a few possible hypotheses, and following up with more observation to figure out which is correct. It's a wonderful example of an international collaboration between telescopes, both in orbit and on the ground, working together to explore and explain the phenomena we see throughout the cosmos."
• February 2, 2018: ESA’s XMM-Newton has spotted surprising changes in the powerful streams of gas from two massive stars, suggesting that colliding stellar winds don’t behave as expected. 98)
- Massive stars – several times larger than our Sun – lead turbulent lives, burning their nuclear fuel rapidly and pouring large amounts of material into their surroundings throughout their short but sparkling lives.
- These fierce stellar winds can carry the equivalent of Earth’s mass in a month and travel at millions of km/hour, so when two such winds collide they unleash enormous amounts of energy. — The cosmic clash heats the gas to millions of degrees, making it shine brightly in X-rays.

Legend to Figure 88: Consisting of two huge stars each 60 times the mass of our Sun, HD 5980 was reported as the first stellar system with colliding winds to be discovered beyond our Milky Way galaxy in 2007. It is located in the Small Magellanic Cloud. The XMM-Newton data collected between 2000 and 2005 show a bright and energetic X-ray source, with variations characteristic of an ongoing interaction between the winds blown by the two stars. The system was expected to gently fade out over the years, but further observations performed with XMM-Newton in 2016 revealed the opposite: the system was two and a half times brighter than it had appeared a decade earlier, and its X-ray emission had shifted to higher energies.
- Normally, colliding winds change little because neither do the stars nor their orbits. However, some massive stars behave dramatically.
- This is the case with HD 5980, a pairing of two huge stars each 60 times the mass of our Sun and only about 100 million km apart – closer than we are to our star.
- One had a major outburst in 1994, reminiscent of the eruption that turned Eta Carinae into the second brightest star in the sky for about 18 years in the 19th century.
- While it is now too late to study Eta Carinae’s historic eruption, astronomers have been observing HD 5980 with X-ray telescopes to study the hot gas.
- In 2007, Yaël Nazé of the University of Liège, Belgium, and her colleagues discovered the collision of winds from these stars using observations made by ESA’s XMM-Newton and NASA’s Chandra X-ray telescopes between 2000 and 2005.
- Then they looked at it again with XMM-Newton in 2016. — “We expected HD 5980 to fade gently over the years as the erupting star settled back to normal – but to our surprise it did just the opposite,” says Yaël.
- They found the pair was two and a half times brighter than a decade earlier, and its X-ray emission was even more energetic.
- “We had never seen anything like that in a wind–wind collision.”
- With less material ejected but more light emitted, it was difficult to explain what was happening. — Finally, they found a theoretical study that offers a fitting scenario.
- “When stellar winds collide, the shocked material releases plenty of X-rays. However, if the hot matter radiates too much light, it rapidly cools, the shock becomes unstable and the X-ray emission dims. - This somewhat counterintuitive process is what we thought happened at the time of our first observations, more than 10 years ago. But by 2016, the shock had relaxed and the instabilities had diminished, allowing the X-ray emission to rise eventually.”
- These are the first observations that substantiate this previously hypothetical scenario. Yaël’s colleagues are now testing the new result in greater detail through computer simulations.
- “Unique discoveries like this demonstrate how XMM-Newton keeps providing astronomers with fresh material to improve our understanding of the most energetic processes in the Universe,” says Norbert Schartel, XMM-Newton project scientist at ESA.
![Figure 89: A Hubble Space Telescope view of the cluster NGC 346 - the arrow indicates the position of HD 5980 [image credit: NASA, ESA, A. Nota (STScI/ESA)] 99)](https://www.eoportal.org/ftp/satellite-missions/x/XMM-Newton_040722/XMM-Newton_Auto3E.jpeg)
• December 11, 2017: A young massive star that began life around 25 times more massive than our own Sun is shedding shells of material and fast winds to create this dynamic scene captured by ESA’s XMM-Newton (Figure 91). 101)
- The star will likely end its life in a violent supernova explosion.
- The Crescent Nebula sits in the constellation of Cygnus about 5000 light-years away, exactly at a location in the sky that has not been accessible to XMM-Newton until recently. Although it has been well studied by other X-ray telescopes, astronomers working on XMM-Newton, which was launched on 10 December 1999, had to wait patiently until the orbit of the satellite was such that this patch of sky moved into its field of view in April 2014.
- More information about XMM-Newton’s observation is available in “X-ray emission from the Wolf-Rayet bubble NGC 688. II. XMM-Newton EPIC observations,” by J. Toalá et al. (2015).

• December 7, 2017: ESA's SPC (Science Program Committee) has approved indicative extensions, up to 2019-2020, for the operation of eight scientific missions. 102)
- During its meeting at ESA Headquarters in Paris, on 21-22 November, the SPC approved indicative extensions for the continued operation of five ESA-led missions: Gaia, INTEGRAL, Mars Express, SOHO, and XMM-Newton. This followed a comprehensive review of the current operational status and outlook of the missions and their expected scientific returns during the extension period. The decision will be subject to confirmation towards the end of 2018.
- The lifetime of Gaia, ESA's billion star surveyor, was extended by eighteen months, from 25 July 2019 to 31 December 2020. This is the first time that Gaia, which was launched in 2013 and originally funded for a five-year mission, has been subject to the extension process. — Mars Express, SOHO, and XMM-Newton each received extensions of two years, so their operations will continue at least until the end of 2020.
- The SPC extended the operations of the high-energy observatory INTEGRAL by one year, until 31 December 2019. A proposal to extend INTEGRAL until the end of 2020, as well as a proposal concerning a two-year extension of the magnetospheric plasma mission, Cluster, will be presented to the next meeting of the SPC in February 2018.
- The go-ahead was also given to continue ESA's contributions to the operations of three international collaborative missions: the HST (Hubble Space Telescope), and IRIS (Interface Region Imaging Spectrograph), which are both led by NASA, as well as the Japanese-led mission Hinode.
- Every two years, all missions whose approved operations end within the following four years are subject to review by the advisory structure of the Science Directorate. Extensions are granted to missions that satisfy the established criteria for operational status and science return, subject to the level of financial resources available in the science program. These extensions are valid for the following four years, subject to a mid-term review and confirmation after two years. Extensions for operations in the period 2017-2018 were approved by the SPC in November 2016, but the indicative extension, for 2019-2020, had been deferred until the November 2017 meeting to allow the SPC to evaluate the outcome of the ESA Ministerial Council meeting in December 2016.
• October 30, 2017: ESA and NASA space telescopes have revealed that, unlike Earth’s polar lights, the intense auroras seen at Jupiter’s poles unexpectedly behave independently of one another. 103)
- Auroras have been seen in many places, from planets and moons to stars, brown dwarfs and a variety of other cosmic bodies. These beautiful displays are caused by streams of electrically charged atomic particles – electrons and ions – colliding with the atmospheric layers surrounding a planet, moon or star. Earth’s polar lights tend to mirror one another: when they brighten at the North pole, they generally brighten at the South pole, too.
- The same was expected of auroras elsewhere, but a new study, published today in Nature Astronomy, reveals that those at the gas giant Jupiter are much less coordinated.
- The study used ESA’s XMM-Newton and NASA’s Chandra X-ray space observatories to observe the high-energy X-rays produced by the auroras at Jupiter’s poles. While the southern auroras were found to pulse consistently every 11 minutes, those at the planet’s north pole flared chaotically. 104)
- “These auroras don’t seem to act in unison like those that we’re often familiar with here on Earth,” says lead author William Dunn of University College London’s MSSL (Mullard Space Science Laboratory), UK, and Harvard-Smithsonian Center for Astrophysics, USA.
- "We thought the activity would be coordinated through Jupiter's magnetic field, but the behavior we found is really puzzling. It's stranger still considering that Saturn – another gas giant planet – doesn't produce any X-ray auroras that we can detect, so this throws up a couple of questions that we're currently unsure how to answer. Firstly, how does Jupiter produce bright and energetic X-ray auroras at all when its neighbor doesn't, and secondly, how does it do so independently at each pole?"
- With the data at hand, William Dunn and colleagues identified and mapped X-ray hot spots at Jupiter's poles. Each hot spot covers an area half the size of Earth's surface.
- As well as raising questions about how auroras are produced throughout the cosmos, Jupiter's independently pulsing auroras suggest that there is far more to understand about how the planet itself produces some of its most energetic emissions.
- Jupiter's magnetic influence is colossal; the region of space over which the Jovian magnetic field dominates – the magnetosphere – is some 40 times larger than Earth's, and filled with high-energy plasma. In the outer edges of this region, charged particles ultimately from volcanic eruptions on Jupiter's moon, Io, interact with the magnetic boundary between the magnetosphere and interplanetary space. These interactions create intense phenomena, including auroras.
- "Charged particles have to hit Jupiter's atmosphere at exceptionally fast speeds in order to generate the X-ray pulses that we've seen. We don't yet understand what processes cause this, but these observations tell us that they act independently in the northern and southern hemispheres," adds Licia Ray, from Lancaster University, UK, and a co-author.
- The asymmetry in Jupiter's northern and southern lights also suggests that many cosmic bodies that are known to experience auroras – exoplanets, neutron stars, brown dwarfs and other rapidly-rotating bodies – might produce a very different aurora at each pole.
- Further studies of Jupiter's auroras will help to form a clearer picture of the phenomena produced at Jupiter; auroral observing campaigns are planned for the next two years, with X-ray monitoring by XMM-Newton and Chandra and simultaneous observations from NASA's Juno, a spacecraft that started orbiting Jupiter in mid-2016.
- ESA's JUICE (JUpiter ICy moons Explorer) will arrive at the planet by 2029, to investigate Jupiter's atmosphere and magnetosphere. It, too, will observe the auroras and in particular the effect on them of the Galilean moons.
- "This is a breakthrough finding, and it couldn't have been done without ESA's XMM-Newton," adds Norbert Schartel, ESA project scientist for XMM-Newton. "The space observatory was critical to this study, providing detailed data at a high spectral resolution such that the team could explore the vibrant colors of the auroras and figure out details about the particles involved: if they're moving fast, whether they're an oxygen or sulphur ion, and so on. Coordinated observations like these, with telescopes such as XMM-Newton, Chandra and Juno working together, are key in exploring and further understanding environments and phenomena across the Universe, and the processes that produce them."

Legend to Figure 92: Jupiter experiences intense auroras that rage constantly, created as charged particles from the Sun and the Jovian moon Io stream towards the gas giant and interact with its atmosphere. - A new study has revealed that the planet's northern and southern lights behave and pulse independently of one another–an unexpected finding given the behavior of Earth's auroras, which tend to mirror one another (when they brighten at the North pole, they generally brighten at the South pole too). - This image, from the NASA/ESA Hubble Space Telescope, shows such an aurora on Jupiter. It is a composite of two separate sets of Hubble observations–one of the polar aurora itself, photographed in the ultraviolet in June 2016, and an optical view of the full disc of Jupiter, taken in April 2014.
• September 6, 2017: A new study using data from NASA's Chandra X-ray Observatory and ESA's XMM-Newton suggests X-rays emitted by a planet's host star may provide critical clues to just how hospitable a star system could be. A team of researchers looked at 24 stars similar to the Sun, each at least one billion years old, and how their X-ray brightness changed over time. 105) 106) 107)
- High levels of magnetic activity can produce bright X-rays and ultraviolet light from stellar flares. Strong magnetic activity can also generate powerful eruptions of material from the star's surface. Such energetic radiation and eruptions can impact planets and could damage or destroy their atmospheres, as pointed out in previous studies, including Chandra work reported in 2011 and 2013.
- Since stellar X-rays mirror magnetic activity, X-ray observations can tell astronomers about the high-energy environment around the star. In the new study the X-ray data from Chandra and XMM-Newton revealed that stars like the Sun and their less massive cousins calm down surprisingly quickly after a turbulent youth.
- “This is good news for the future habitability of planets orbiting Sun-like stars, because the amount of harmful X-rays and ultraviolet radiation striking these worlds from stellar flares would be less than we used to think,” said Rachel Booth, a graduate student at Queen’s University in Belfast, UK, who led the study.
- To understand how quickly stellar magnetic activity level changes over time, astronomers need accurate ages for many different stars. This is a difficult task, but new precise age estimates have recently become available from studies of the way that a star pulsates using NASA’s Kepler and ESA’s CoRoT missions. These new age estimates were used for most of the 24 stars studied here.

Legend to Figure 93: This artist's illustration depicts one of these comparatively calm, older Sun-like stars with a planet in orbit around it. The large dark area is a "coronal hole", a phenomenon associated with low levels of magnetic activity. The inset box shows the Chandra data of one of the observed objects, a two billion year old star called GJ 176, located 30 light years from Earth.
• July 10, 2017: Highly obscured and rapidly growing SMBH (Super-Massive Black Holes), known as AGN (Active Galactic Nuclei), might represent the key phase when SMBH accreted most of their mass and when the relationship between galaxies and their central SMBHs was established. A new study by an international team of astronomers led by Silvia Mateos from the Instituto de Física de Cantabria (CSIC-UC) in Spain, now suggests that many of the brightest SMBH may be escaping our detection as they hide in heavily obscured environments. 108)
- A large observational effort has been devoted to reveal the obscured AGN phase across the history of the Universe. Oddly enough, most AGN searches, mainly at X-ray wavelengths, report that obscured AGN (optically classified as type 2) become rarer with increasing accretion power. The receding torus model hypothesis is often invoked to explain this lack of luminous highly obscured AGN. It postulates that the most luminous AGN erode the obscuring material located in the vicinity of the SMBH, the so-called "dusty torus", reducing its covering factor. As a result, higher luminosity AGN should be rarely hidden from our view by the dusty torus.
- A completely different picture emerges now. Based on observations from both ground and space instruments, including spectroscopic observations with ISIS and ACAM on the William Herschel Telescope (WHT) and the European Photon Imaging Camera onboard the XMM-Newton observatory, the team led by Mateos built a sample of approximately 200 X-ray selected AGN from the Bright Ultra-hard XMM-Newton Survey (BUXS). Using models that self-consistently reproduce the emission from dust in the torus heated by the AGN, they determined for each AGN in their sample the fraction of the sky around the AGN central engine that is obscured, i.e., the geometrical covering factor of the dusty torus. The combined torus covering factor over the entire AGN population is precisely the intrinsic fraction of type 2 AGN.
- By equating the distribution of torus covering factors to the observed fraction of type 2 AGN in BUXS the team finds that many type 2 AGN with high covering factor tori have escaped detection in X-rays. Most importantly, these elusive AGN are increasingly numerous at higher AGN luminosities. When they account for the "missing" AGN, the team finds an intrinsic fraction of type 2 AGN of 58% with no (or at most a weak) dependence with AGN luminosity over three orders of magnitude in luminosity.
- According to Silvia Mateos, who led the research: "Clearly, the impact of the enormous AGN accretion power on the geometry of the nuclear material surrounding the SMBH is not as strong as we thought. Instead, a large fraction of luminous rapidly growing SMBH are so deeply embedded that they are escaping X-ray detection". 109)

• June 6, 2017: This blue ‘ball of strings’ actually records 2114 movements made by ESA’s XMM-Newton space telescope as it shifted its gaze from one X-ray object to another between August 2001 and December 2014 (Figure 95). — Orbiting in space since 1999, XMM-Newton is studying high-energy phenomena in the Universe, such as black holes, neutron stars, pulsars and stellar winds. 110)
- Even when moving its focus between objects, the space telescope collects scientific data, revealing X-ray sources across the entire sky. After correcting for overlaps between slews, 84% of the sky has now been covered.
- The plot is in galactic coordinates such that the center of the plot corresponds to the center of the Milky Way. The slew paths pass predominantly through the ecliptic poles, indicated by the density of overlapping slew paths to the top left and bottom right.
- Over 5000 papers have been published on XMM-Newton results to date. Scientists are also looking forward to the next generation of X-ray satellite, such as ESA’s ATHENA (Advanced Telescope for High-ENergy Astrophysics), which is expected to be launched towards the end of the next decade.

• May 15, 2017: This colorful, seemingly abstract artwork is actually a map (Figure 96) depicting all the celestial objects that were detected in the XMM-Newton slew survey between August 2001 and December 2014. 111)
- Orbiting Earth since 1999, XMM-Newton is studying high-energy phenomena in the Universe, such as black holes, neutron stars, pulsars and stellar winds. But even when moving between specific targets, the space telescope collects scientific data.
- The plot is in galactic coordinates such that the center of the plot corresponds to the center of the Milky Way. High-energy sources along the center of the Milky Way include the famous black hole Cygnus X-1, and Vela X-1, a binary system comprising a neutron star consuming matter from a supergiant companion.
- Several star-and-black hole binary systems are also captured, including objects identified as GRS 1915+105, 4U 1630-47 and V 4641 Sgr.
- Two clusters of sources, one to the top left and one to the bottom right, correspond to the ecliptic poles. Objects above and below the plane of our Galaxy are predominantly external galaxies that are emitting X-rays from their massive black holes.

• May 10, 2017, Astronomers produced this dramatic new, highly-detailed image of the Crab Nebula (Figure 97) by combining data from telescopes spanning nearly the entire breadth of the electromagnetic spectrum, from the long waves seen by the Karl G. Jansky VLA (Very Large Array) to the extremely short waves seen by the orbiting Chandra X-Ray Observatory. 112) 113)
- The Crab Nebula, the result of a bright supernova explosion seen by Chinese and other astronomers in the year 1054, is some 6,500 light-years from Earth. At its center is a superdense neutron star, rotating once every 33 milliseconds, shooting out rotating lighthouse-like beams of radio waves and light — a pulsar. The nebula’s intricate shape is caused by a complex interplay of the pulsar, a fast-moving wind of particles coming from the pulsar, and material originally ejected by the supernova explosion and by the star itself before the explosion.
- The new VLA, Hubble, and Chandra observations all were made at nearly the same time in November of 2012. A team of scientists led by Gloria Dubner of IAFE, the National Council of Scientific Research (CONICET), and the University of Buenos Aires in Argentina then made a detailed analysis of the newly-revealed details in a quest to gain new insights into the complex physics of the object. They are reporting their findings in the Astrophysical Journal. 114)
- New details from the study show interactions between fast-moving particles and magnetic fields similar to structures seen on the Sun, other features seen to appear at multiple wavelengths, and structures that may indicate features near the star before it exploded. Two separate jets of material from near the pulsar appear in the X-ray and the radio images.
- “Comparing these new images, made at different wavelengths, is providing us with a wealth of new detail about the Crab Nebula. Though the Crab has been studied extensively for years, we still have much to learn about it,” Dubner said.
- The NRAO (National Radio Astronomy Observatory) is a facility of the NSF (National Science Foundation), operated under cooperative agreement by Associated Universities, Inc.

• March 1, 2017: ESA and NASA space telescopes have made the most detailed observation of an ultra-fast wind flowing from the vicinity of a black hole at nearly a quarter of the speed of light. Outflowing gas is a common feature of the supermassive black holes that reside in the center of large galaxies. Millions to billions of times more massive than the Sun, these black holes feed off the surrounding gas that swirls around them. Space telescopes see this as bright emissions, including X-rays, from the innermost part of the disc around the black hole. 115)
- Occasionally, the black holes eat too much and burp out an ultra-fast wind. These winds are an important characteristic to study because they could have a strong influence on regulating the growth of the host galaxy by clearing the surrounding gas away and therefore suppressing the birth of stars.
- Using ESA’s XMM-Newton and NASA’s NuSTAR telescopes, scientists have now made the most detailed observation yet of such an outflow, coming from an active galaxy identified as IRAS 13224–3809. The winds recorded from the black hole reach 71 000 km/s – 0.24 times the speed of light – putting it in the top 5% of fastest known black hole winds.
- XMM-Newton focused on the black hole for 17 days straight, revealing the extremely variable nature of the winds. “We often only have one observation of a particular object, then several months or even years later we observe it again and see if there’s been a change,” says Michael Parker of the Institute of Astronomy at Cambridge, UK, lead author of the paper published in Nature this week that describes the new result. ”Thanks to this long observation campaign, we observed changes in the winds on a timescale of less than an hour for the first time.” 116)
- The changes were seen in the increasing temperature of the winds, a signature of their response to greater X-ray emission from the disc right next to the black hole.
- Furthermore, the observations also revealed changes to the chemical fingerprints of the outflowing gas: as the X-ray emission increased, it stripped electrons in the wind from their atoms, erasing the wind signatures seen in the data. “The chemical fingerprints of the wind changed with the strength of the X-rays in less than an hour, hundreds of times faster than ever seen before,” says co-author Andrew Fabian, also from the Institute of Astronomy and principal investigator of the project. “It allows us to link the X-ray emission arising from the infalling material into the black hole, to the variability of the outflowing wind farther away.”
- “Finding such variability, and finding evidence for this link, is a key step in understanding how black hole winds are launched and accelerated, which in turn is an essential part of understanding their ability to moderate star formation in the host galaxy,” adds Norbert Schartel, ESA’s XMM-Newton project scientist.
- The brightness of an active galactic nucleus is set by the gas falling onto it from the galaxy, and the gas infall rate is regulated by the brightness of the active galactic nucleus; this feedback loop is the process by which supermassive black holes in the centers of galaxies may moderate the growth of their hosts.

• February 21, 2017: ESA’s XMM-Newton has found a pulsar – the spinning remains of a once-massive star – that is a thousand times brighter than previously thought possible (Figure 99). The pulsar is also the most distant of its kind ever detected, with its light travelling 50 million light-years before being detected by XMM-Newton. 117) 118)
- Pulsars are spinning, magnetized neutron stars that sweep regular pulses of radiation in two symmetrical beams across the cosmos. If suitably aligned with Earth these beams are like a lighthouse beacon appearing to flash on and off as it rotates. They were once massive stars that exploded as a powerful supernova at the end of their natural life, before becoming small and extraordinarily dense stellar corpses.
- This X-ray source is the most luminous of its type detected to date: it is 10 times brighter than the previous record holder. In one second it emits the same amount of energy released by our Sun in 3.5 years.
- XMM-Newton observed the object several times in the last 13 years, with the discovery a result of a systematic search for pulsars in the data archive – its 1.13 s periodic pulses giving it away. The signal was also identified in NASA’s NuSTAR archive data, providing additional information.
- “Before, it was believed that only black holes at least 10 times more massive than our Sun feeding off their stellar companions, could achieve such extraordinary luminosities, but the rapid and regular pulsations of this source are the fingerprints of neutron stars and clearly distinguish them from black holes,” says Gian Luca Israel, from the INAF-Osservatorio Astronomica di Roma, Italy, lead author of the paper describing the result published in Science this week.
- The archival data also revealed that the pulsar’s spin rate has changed over time, from 1.43 s per rotation in 2003 to 1.13 s in 2014. The same relative acceleration in Earth’s rotation would shorten a day by five hours in the same time span. “Only a neutron star is compact enough to keep itself together while rotating so fast,” adds Gian Luca.
- Although it is not unusual for the rotation rate of a neutron star to change, the high rate of change in this case is likely linked to the object rapidly consuming mass from a companion. “This object is really challenging our current understanding of the ‘accretion’ process for high-luminosity stars,” says Gian Luca. “It is 1000 times more luminous than the maximum thought possible for an accreting neutron star, so something else is needed in our models in order to account for the enormous amount of energy released by the object.”
- The scientists think there must be a strong, complex magnetic field close to its surface, such that accretion onto the neutron star surface is still possible while still generating the high luminosity.
- “The discovery of this very unusual object, by far the most extreme ever discovered in terms of distance, luminosity and rate of increase of its rotation frequency, sets a new record for XMM-Newton, and is changing our ideas of how such objects really ‘work’,” says Norbert Schartel, ESA’s XMM-Newton project scientist.
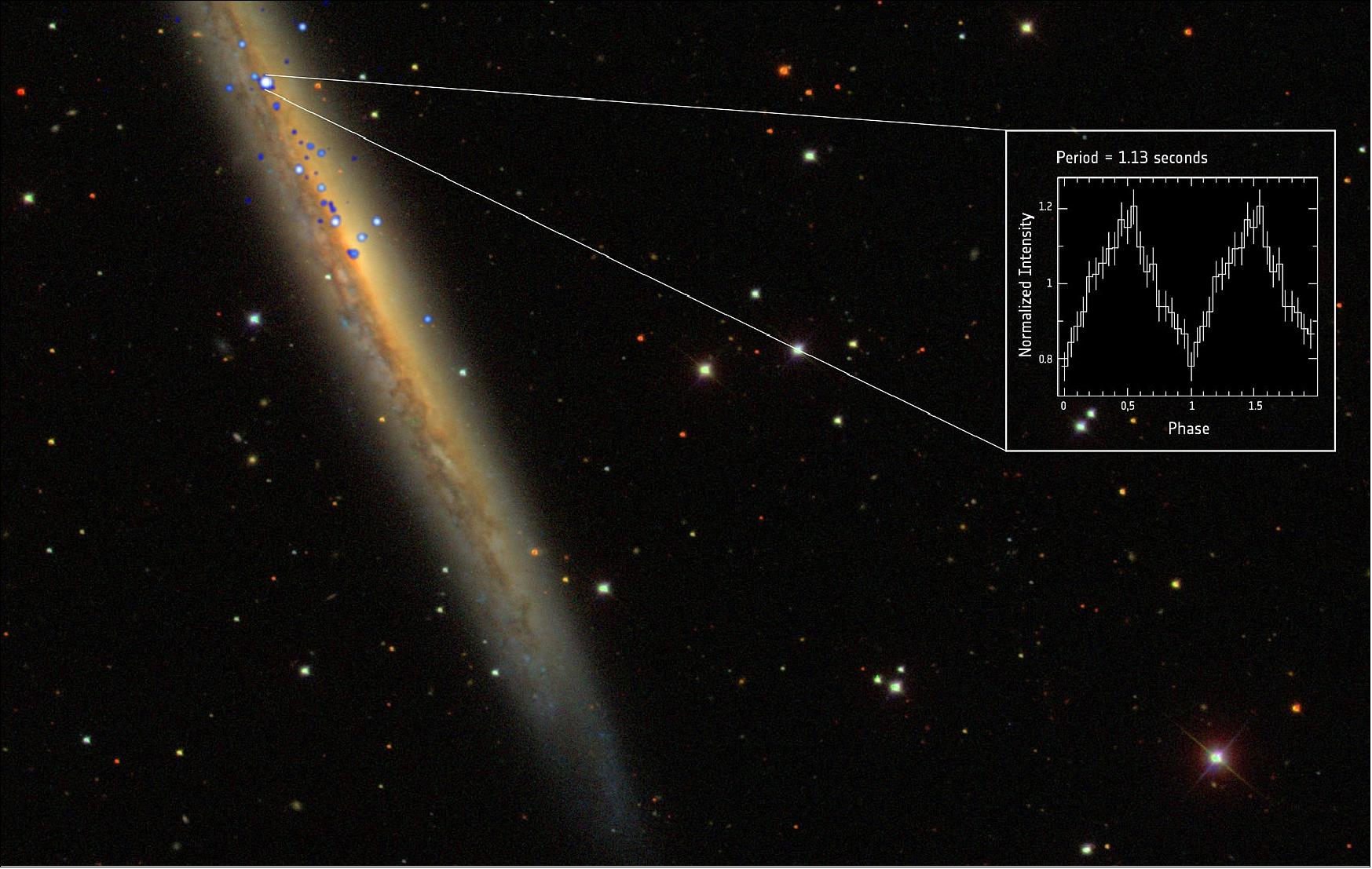
Legend to Figure 99: The inset shows the X-ray pulsation of the spinning neutron star, which has a period of 1.13 s, as determined by XMM-Newton’s European Photon Imaging Camera.
• January 31, 2017: Scientists observing a curious neutron star in a binary system known as the 'Rapid Burster' may have solved a forty-year-old mystery surrounding its puzzling X-ray bursts. They discovered that its magnetic field creates a gap around the star, largely preventing it from feeding on matter from its stellar companion. Gas builds up until, under certain conditions, it hits the neutron star all at once, producing intense flashes of X-rays. The discovery was made with space telescopes including ESA's XMM-Newton. 119)
- Discovered in the 1970s, the Rapid Burster is a binary system comprising a low-mass star in its prime and a neutron star – the compact remnant of a massive star's demise. In such a stellar pair, the gravitational pull of the dense remnant strips the other star of some of its gas; the gas forms an accretion disc and spirals towards the neutron star.
- As a result of this accretion process, most neutron star binaries continuously release large amounts of X-rays, which are punctuated by additional X-ray flashes every few hours or days. Scientists can account for these 'type-I' bursts, in terms of nuclear reactions that are ignited in the inflowing gas – mainly hydrogen – when it accumulates on the neutron star's surface.
- But the Rapid Burster is a peculiar source: at its brightest, it does emit these type-I flashes, while during periods of lower X-ray emission, it exhibits the much more elusive 'type-II' bursts – these are sudden, erratic and extremely intense releases of X-rays.
- In contrast to type-I bursts, which do not represent a significant release of energy with respect to what is normally emitted by the accreting neutron star, bursts of type-II liberate enormous amounts of energy during periods otherwise characterized by very little emission occurring (the relative energy output of a burst with respect to the normal accretion process is tens to hundreds of times higher in type-II bursts than in type-I bursts).
- Despite forty years of searches, type-II bursts have been detected only in one other source besides the Rapid Burster. Known as the Bursting Pulsar and discovered in the 1990s, this binary system comprises a low-mass star and a highly magnetized, spinning neutron star – a pulsar – that exhibits only type-II bursts.
- Because of the scarcity of sources that display this phenomenon, the underlying physical mechanisms have long been debated, but a new study of the Rapid Burster provides first evidence for what is occurring.
- "The Rapid Burster is the archetypal system to investigate type-II bursts – it's where they were first observed and the only source that shows both type-I and type-II bursts," says Jakob van den Eijnden, a PhD student at the Anton Pannekoek Institute for Astronomy in Amsterdam, The Netherlands, and lead author of a Letter published in Monthly Notices of the Royal Astronomical Society. 120) — In this study, Jakob and his colleagues organized an observing campaign using three X-ray space telescopes to find out more about this system.
- Under the coordination of co-author Tullio Bagnoli, who was also based at the Anton Pannekoek Institute for Astronomy, the team managed to observe the source bursting over a few days in October 2015 with a combination of NASA's NuSTAR and Swift, and ESA's XMM-Newton.
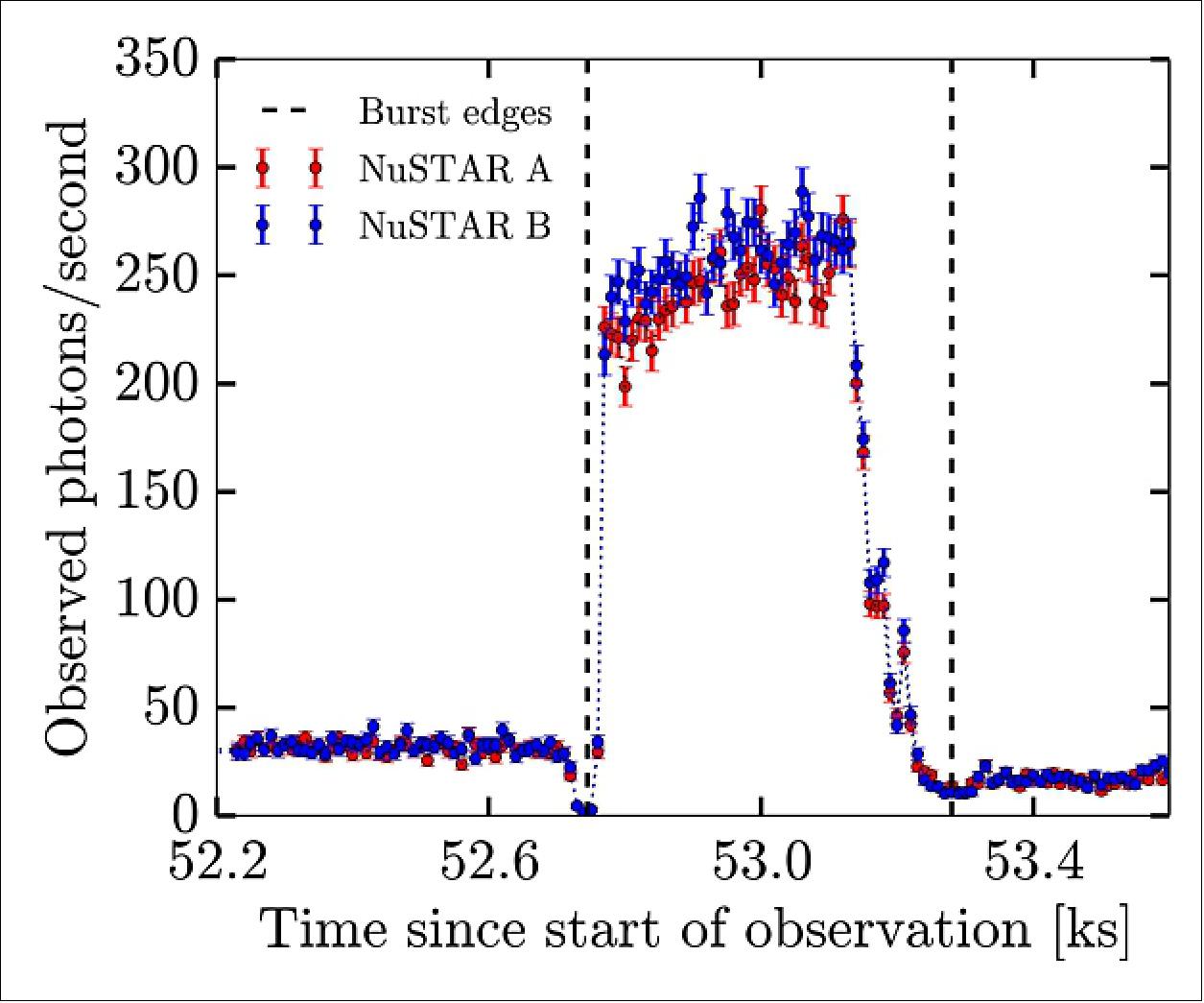
- They first monitored the source with Swift, timing the observations for a period when they expected a series of type-II bursts to take place. Then, soon after the first burst was detected, the scientists set the other observatories into motion, using XMM-Newton to measure X-rays emitted directly by the neutron star's surface or by gas in the accretion disc, and NuSTAR to detect higher-energy X-rays, which are emitted by the neutron star and reflected off the disc. - With these data, the scientists scrutinized the structure of the accretion disc to understand what happens to it before, during, and after these copious releases of X-rays.
- According to one model, type-II bursts occur because the fast spinning magnetic field of the neutron star keeps the gas flowing from the companion star at bay, preventing it from reaching closer to the neutron star and effectively creating an inner edge at the center of the disc. However, as the gas continues to flow and accumulate near this edge, it spins faster and faster, and eventually catches up with the spinning velocity of the magnetic field.
- "It's as if we threw something towards a merry-go-round that is spinning very fast: it would bounce off, unless it's thrown at the same velocity as the machine," explains Jakob. "A similar balancing act happens between the inflowing gas and the spinning magnetic field: as long as the gas hasn't the right speed, it cannot get to the neutron star and it can only pile up at the edge. By the time it reaches the right velocity, a lot of gas has accumulated and it hits the neutron star all at once, giving rise to the dramatic emission of type-II bursts."
- This model predicts that, while the material is piling up, a gap should form between the neutron star and the edge of the accretion disc.

- In other models, the intense flashes are explained as arising from instabilities in the flow of the accreting gas or from general-relativistic effects. In either case, these would take place much closer to the neutron star and not give rise to such a gap. "A gap is exactly what we found at the Rapid Burster," says Nathalie Degenaar, a researcher at Anton Pannekoek Institute for Astronomy and Jakob's PhD advisor. "This strongly suggests that the type-II bursts are caused by the magnetic field."
- The observations indicate that there is a gap of roughly 90 km between the neutron star and the inner edge of the accretion disc. While not impressive on cosmic scales, the size of the gap is much larger than the neutron star itself, which has a radius of about 10 km.
- This finding is in line with results from a previous study by Nathalie and collaborators, who had observed a similar gap around the Bursting Pulsar – the other source known to produce type-II bursts.
- In the new study of the Rapid Burster, the scientists also measured the strength of the neutron star's magnetic field: at 6 x 108 G (gauss), it is around a billion times stronger than Earth's and, most important, over five times stronger than observed in other neutron stars with a low-mass stellar companion. This could hint at a young age of this binary system, suggesting that the accretion process has not been going on for long enough to damp the magnetic field down, as is thought to have happened in similar systems.
- If this neutron star binary really is as young as its strong magnetic field indicates, then it is expected to spin much slower than its older counterparts: future measurements of the star's spinning rate might help confirm this unusual scenario.
- "This result is a big step towards solving a forty-year-old puzzle in neutron star astronomy, while also revealing new details about the interaction between magnetic fields and accretion discs in these exotic objects," concludes Norbert Schartel, XMM-Newton Project Scientist at ESA.
• December 19, 2016: An important part of studying celestial objects is understanding and removing the background noise. The image presented in Figure 102 was created to demonstrate the power of software tools used to analyze observations by ESA’s XMM-Newton of large objects like galaxies, clusters of galaxies, and supernova remnants. The tool models and subtracts the background noise, which is very difficult for large, fuzzy objects like these, and to create exposure-corrected images. It also merges and smooths the observations taken of an individual object by XMM-Newton’s three X-ray cameras of the EPIC (European Photon Imaging Camera) instrument, and to allow the mosaicking of multiple observations. 122)
- It certainly does not look like it, but the star-like feature on the right corresponds to spiral galaxy M81. Similarly, the feature on the left arises from the Holmberg IX dwarf galaxy.
- By examining images like these, along with complementary images taken at other wavelengths, scientists can get a quick look at the structure of the object and the spectral variations across the field. The structure of the bright patterns contains information about the origin of the emission, such as whether it comes from a ‘halo’ around the galaxy, or is confined to the disc and arms.
- For example, this particular image shows that there is a bright point source at the center of M81, resulting from the galaxy’s active core. There is also a decrease of brightness away from the central source, with fainter extended emission around it. Another galaxy might display brighter emission along its spiral arms.
- Bright emission is also apparent from the X-ray source in the dwarf galaxy. The ‘rays’ extending from the point sources are artefacts, seen whenever there is a very bright point source in the field of view. But even artefacts can be beautiful.
- The XMM-Newton Extended Source Analysis Software package (XMM-ESAS) was developed at the NASA/GSFC ( Goddard Space Flight Center) XMM-Newton GOF (Guest Observer Facility) in cooperation with the XMM-Newton Science Operations Center and the Background Working Group.

• December 8, 2016: XMM-Newton is one of Europe’s longest-flying and most productive orbiting observatories, investigating the hot X-ray Universe. Thanks to teamwork and technical innovation, it’s on track to keep flying for a long time yet. Launched 17 years ago, ESA’s orbiting X-ray telescope has helped scientists around the world to understand some of our Universe’s most mysterious events, from what happens in and around black holes to how galaxies formed. At 3800 kg, the 10 m-long XMM-Newton is the biggest science satellite ever built in Europe and its telescope mirrors are the most sensitive ever developed.-Expected to operate for as long as a decade, the hardy spacecraft has happily surprised everyone by lasting almost two decades – and it shows no signs of giving up. 123)
- The success of XMM-Newton has been possible not only because of the robust spacecraft, but also the close cooperation between ESA’s astronomy center near Madrid, Spain, and the mission controllers at ESA’s operations center in Darmstadt, Germany.
- “The total number of 4775 scientific publications to date, with 358 from this year alone, is an impressive record of the mission’s scientific success, covering many, many areas of astrophysics,” notes project scientist Norbert Schartel.
- But keeping it fit and healthy into its third decade means the team must continue to develop and test new control techniques. A complex change to the orbit control system has almost halved fuel consumption, for example.
- (Not) running on empty: For starters, keeping XMM in orbit will require occasional thruster firings, about once per day, and that means burning fuel. “We’ve got plenty of fuel and over the years we’ve figured out how to use less and less to maintain our science orbit,” says Marcus Kirsch, spacecraft operations manager. “The fuel is distributed between four separate tanks, but the main tank will run dry first. The design means we could not use the remaining fuel in the other tanks, so we're moving it all into tank 1. This will enable us to continue operations into the coming decade.”
- Back in the control room: As part of this process, the flight control team returned to ESA’s large, general-purpose Main Control Room at mission control in November – the first time since launch in 1999 – for five days of intensive simulations. The team usually works from a smaller, dedicated room shared with the Integral and Gaia mission teams. — The simulations checked the procedures that will be used for moving the fuel and for reconfiguring XMM for working beyond 2017.
- No one's ever done this before: “Not many spacecraft use the specially designed tank fuel system like on XMM,” says Nikolai von Krusenstiern, spacecraft operations engineer. - “As far as we know, no one’s ever shifted fuel from one tank to another with a tank design like ours in a satellite in orbit, and we want to take as much time as necessary to minimize any risk to the mission.” Tank-to-tank replenishment was never foreseen in the original design specifications – as XMM wasn’t meant to last so long – so no process was devised by builder Astrium (now Airbus Defence & Space). “Airbus have been very helpful – they even helped us get in touch with the now-retired designer of the fuel system to help us to safely design the procedures,” says Nikolai.
- XMM's third decade: The team will now analyze results of last month’s simulations with the aim of reconfiguring the spacecraft in 2017. This will complement the careful optimization of the flight control procedures already in place, and keep XMM thrusters firing – and the spacecraft flying reliably – into 2023. After that, the team will have a low-risk and confirmed plan on hand to conduct the fuel replenishment, which would thereafter keep the craft in its science mission well into its third decade. “The time spent in training and simulations last month was hugely valuable for the entire team,” says Marcus. “We worked together to devise a solid solution for XMM’s coming decades, and the individual engineers gained excellent training experience that they can use for XMM or even take with them if assigned to other missions.”
• December 8, 2016: XMM-Newton delivers a selfie to mark 17 years of X-ray science success. Launched on 10 December 1999, XMM-Newton is an X-ray observatory designed to investigate some of the most violent phenomena in the Universe. Sources that emit large amounts of X-rays include remnants of supernova explosions and the surroundings of black holes. — Detecting this energetic radiation is a daunting endeavor, requiring techniques that are greatly different from those used in traditional telescopes. In the case of XMM-Newton, it carries three telescopes of 58 nested mirrors each. These sit at one end of a 7 m-long tube, while at the other end are the scientific instruments at the focus. 124)
- The cameras were originally used by controllers to check how the solar wings unfolded after launch, and have remained dormant since 2003.


- In the image on the left, one camera captured the Sun side of one of XMM’s solar wings (at left in the image), and the dark multilayer insulation on the service module, the bright Sun-shielding behind and a dark box-like structure topped by a pair of thrusters (at right in the image).
- In the image on the right, the other camera captured the dark tripod of the S-band antenna (at left in the image) and then the 2A/2B thruster pair (at center) and XMM’s other solar wing (at right).
• November 22, 2016: ESA's Science Program Committee (SPC) has today confirmed two-year mission extensions for nine scientific missions in which the Agency is participating. This secures their operations until the end of 2018. — After a comprehensive review of their current operational status and the likely scientific return from each mission, the SPC decided to extend the operation of six ESA-led missions (Cluster, INTEGRAL, Mars Express, PROBA-2, SOHO and XMM-Newton) from 1 January 2017 to 31 December 2018. 125)
- The go-ahead was also given to continue ESA's contributions to the operations of three international collaborative missions: the Hubble Space Telescope and the Interface Region Imaging Spectrograph (IRIS), which are both led by NASA, as well as Solar-B (Hinode), which is a Japanese-led mission.
• August 29, 2016: A giant bubble surrounding the center of the Milky Way shows that six million years ago our Galaxy's supermassive black hole was ablaze with furious energy. It also shines a light on the hiding place of the Galaxy's so-called 'missing' matter. - While the mysterious dark matter grabs most of the headlines, astronomers also know that they have yet to find all of the normal, so-called baryonic, matter in the Galaxy. That has now changed thanks to the work of ESA's X-ray observatory XMM-Newton. 126)
- A thorough analysis of archival observations has shown that there is a vast quantity of baryonic matter scattered through the Galaxy. XMM-Newton found it in the form of gas at a temperature of one million degrees that permeates both the disc of the Galaxy, where the majority of the stars are found, and a spherical volume that surrounds the whole Galaxy. — The spherical cloud is vast. Whereas the Sun lies just 26 000 light years from the center of the Galaxy, the cloud extends out to a distance of at least 200 000 – 650 000 light years.
- Fabrizio Nicastro, from the Istituto Nazionale di Astrofisica, Osservatorio Astronomico di Roma, Italy, and his colleagues have been on the trail of the missing baryons for more than 15 years now. Their latest discovery with XMM-Newton shows that there is enough million-degree-hot gas in the Galaxy to account for it all. 127)
- It has remained undetected for so long because it does not emit visible light. Instead, the astronomers found it because the oxygen in the cloud absorbed X-rays at very specific wavelengths from light being emitted by more distant celestial objects. - And this was not the only discovery waiting in the data for the team. When it came to model the data with computer simulations to understand the way in which the gas was distributed around the Galaxy, the team did not get the answer they were expecting.
- "According to simple gravitational physics you expect the density of the gas to decrease from the center outwards," says Nicastro. In this picture, the density of gas will be at its peak in the center of the Galaxy and at its least on the outer edges. But there was a hitch. "I spent three months trying to match the data with that model and I just couldn't," says Nicastro.
- Having tried everything else, he moved the peak density away from the center of the Galaxy. At a distance of about 20 000 light years from the Galaxy's center the model fitted better. It was puzzling why this should improve things until he remembered that this distance is also the size of two large 'balloons' of gamma rays found in 2010 by NASA's Fermi gamma-ray observatory, which extend tens of thousands of light-years above and below the center of our Galaxy.
- So Nicastro constructed a different density model, in which there was a central bubble of low density gas extending out to 20 000 light years. When he applied this model to his X-ray data, he found that it fitted excellently. "That was unexpected and very exciting at the same time," says Nicastro. It meant that something had pushed the gas outwards from the center of the Galaxy, creating a giant bubble.

• July 12, 2016: ESA's orbiting X-ray observatory, XMM-Newton, has proved the existence of a 'gravitational vortex' around a black hole. The discovery, aided by NASA's NuSTAR mission, solves a mystery that has eluded astronomers for more than 30 years and will allow them to map the behavior of matter very close to black holes. It could also open the door to future investigations of Albert Einstein's general relativity. 129) 130) 131)
- Matter falling into a black hole heats up as it plunges to its doom. Before it passes into the black hole and is lost from view forever, it can reach millions of degrees. At that temperature it shines X-rays into space.
- In the 1980s, pioneering astronomers using early X-ray telescopes discovered that the X-rays coming from black holes flicker. The changes follow a set pattern. When the flickering begins, the dimming and re-brightening can take 10 seconds to complete. As the days, weeks and then months progress, the period shortens until the oscillation takes place 10 times every second. Then, the flickering suddenly stops altogether.
- The phenomenon was dubbed the QPO (Quasi Periodic Oscillation). "It was immediately recognized to be something fascinating because it is coming from something very close to a black hole," says Adam Ingram, University of Amsterdam, The Netherlands, who began working to understand QPOs for his PhD in 2009. — During the 1990s, astronomers had begun to suspect that the QPOs were associated with a gravitational effect predicted by Einstein's general relativity: that a spinning object will create a kind of gravitational vortex.
- "It is a bit like twisting a spoon in honey. Imagine that the honey is space and anything embedded in the honey will be 'dragged' around by the twisting spoon," explains Ingram. "In reality, this means that anything orbiting a spinning object will have its motion affected." In the case of an inclined orbit, it will 'precess'. This means that the whole orbit will change orientation around the central object. The time for the orbit to return to its initial condition is known as a precession cycle.
- In 2004, NASA launched Gravity Probe B to measure this so-called Lense-Thirring effect around Earth. After painstaking analysis, scientists confirmed that the spacecraft would turn through a complete precession cycle once every 33 million years.
- Around a black hole, however, the effect would be much more noticeable because of the stronger gravitational field. The precession cycle would take just a matter of seconds or less to complete. This is so close to the periods of the QPOs that astronomers began to suspect a link.
- Ingram began working on the problem during his PhD, looking at what happened in the flat disc of matter surrounding a black hole. Known as an accretion disc, it is the place where material gradually spirals inwards towards the black hole. It had already been suggested that, close to the black hole, the flat accretion disc puffs up into a hot plasma, in which electrons are stripped from their host atoms. Termed the hot inner flow, it shrinks in size over weeks and months as it is eaten by the black hole. Together with colleagues, Ingram published a paper in 2009 suggesting that the QPO is driven by Lense-Thirring precession of this hot flow. This is because the smaller the inner flow becomes, the closer to the black hole it would approach and so the faster its Lense-Thirring precession cycle would be. The question was: how to prove it?
- The answer was that the inner flow is releasing high energy radiation that strikes the matter in the surrounding accretion disc, making the iron atoms in the disc shine like a fluorescent light tube. Instead of visible light, the iron releases X-rays of a single wavelength - referred to as 'a line'.
- Because the accretion disc is rotating, the iron line has its wavelength distorted by the Doppler effect. Line emission from the approaching side of the disc is squashed – blue shifted – and line emission from the receding disc material is stretched – red shifted. If the inner flow really is precessing, it will sometimes shine on the approaching disc material and sometimes on the receding material, making the line wobble back and forth over the course of a precession cycle.
- Seeing this wobbling is where XMM-Newton came in. Ingram and colleagues from Amsterdam, Cambridge, Durham, Southampton and Tokyo applied for a long duration observation that would allow them to watch the QPO repeatedly. They chose black hole H 1743-322, which was exhibiting a four-second QPO at the time. They watched it for 260,000 seconds with XMM-Newton. They also observed it for 70,000 seconds with NASA's NuSTAR X-ray observatory.
- After a complicated analysis procedure to add all the observational data together, they saw that the iron line was wobbling in accordance with the predictions of general relativity. "We are directly measuring the motion of matter in a strong gravitational field near to a black hole," says Ingram.
- This is the first time that the Lense-Thirring effect has been measured in a strong gravitational field. The technique will allow astronomers to map matter in the inner regions of accretion discs around black holes. It also hints at a powerful new tool with which to test general relativity.
- Einstein's theory is largely untested in such strong gravitational fields. So if astronomers can understand the physics of the matter that is flowing into the black hole, they can use it to test the predictions of general relativity as never before - but only if the movement of the matter in the accretion disc can be completely understood.
- "This is a major breakthrough since the study combines information about the timing and energy of X-ray photons to settle the 30-year debate around the origin of QPOs. The photon collecting capability of XMM-Newton was instrumental in this work," says Norbert Schartel, ESA Project Scientist for XMM-Newton.

Legend to Figure 106: In these three views, the precessing inner disc shines high energy radiation that strikes the matter in the surrounding accretion disc, causing the iron atoms in that disc to emit in X-rays, depicted as the glow on the accretion disc to the right (in view a), to the front (in view b) and to the left (in view c).
• May 2016: Current technical evaluations suggest that the XMM-Newton spacecraft and its scientific instruments may continue to provide first class X-ray observations well into the next decade. 132)
• May 2016: Improvement of the operational XMM-Newton system for more efficient operations support. The original design of the XMM-Newton mission operations was based on continuous ground station coverage and cannot be changed anymore, since the spacecraft has no mass memory on board. Telecommanding and telemetry reception is therefore handled “live” from the MCS (Mission Control System) with individual commands, sent for routine operations prepared and bundled into an automated timeline. This timeline runs in real time and sends all the required commands. Verification of telecommanding is done by the SPACON (SPAcecraft CONtroller), using SCOS-2000 (Spacecraft Control & Operation System-2000) as mission control system. 133)
- Through an operational change of the AOCS system, the project team extended its potential lifetime until the late 20's of this century. Spacecraft and instruments are performing without major degradation. Because of the highly elliptical orbit, the spacecraft is permanently visible from the ground station network. For this and other reasons, the originally chosen on-board autonomy design is very limited. Fifteen years of inflight experience created a deep understanding of the spacecraft behavior including elaborated strategies and procedures to recover from reoccurring non-critical anomalies on board. Based on this experience, an automated ground failure detection system has been developed to provide reliable fault isolation and adapted reaction.
- The newly implemented on-ground FDIR (Failure Detection, Isolation and Recovery) system is able to read various sorts of anomaly messages, either from the spacecraft (telemetry being out-of-limit), from the ground segment (link loss) or from the mission control software (an application stopped). Upon detection of anomaly, the automation system starts the execution of a procedure to confirm its consistency and persistence to avoid over reaction to minor events. Once the anomaly has been confirmed and identified, the recovery is kicked off under the supervision of the operator or autonomously. The targeted level of automation shall release the operator from activities that were so far manually performed, let him concentrate on other activities running in parallel, reduce the stress in contingency situations and lessen the probability of human error.
- Outlook: The usage of an automation system has shown its relevance on a mission like XMM-Newton so that the flight control team members are stepping forward to include more automated procedures. Among the upcoming challenges, the automation of the ground stations activities is quite promising. Indeed, the spacecraft controller has to coordinate via the voice loop with the operator of ESA’s tracking station network. Being able to control the setup of the TM and TC links would contribute to largely increase the autonomy of operations performed from ground.
- The on-ground FDIR system, called MOIS (Manufacturing and Operation Information System) Event Monitor, has been designed to react to different types of events. The detection relies on collection of known events and results in the trigger of an automated procedure. This procedure checks the consistency of the anomaly using details provided by the mission control system and a MOIS internal database. Assuming that the anomaly is confirmed, the links to the satellite are correctly established and there is no constraining up-coming activity, MOIS requests the start of the contingency recovery procedure. Belonging to a larger automation system, the Event Monitor is a real support for the human controller and facilitates his work during repeating and heavy operations.
- Every contingency recovery procedures that had to be automated was reviewed and streamlined. After such a long time in orbit, this was a good exercise for the flight control team to re-familiarize with the procedures and also a great opportunity to update and increase further the robustness of the procedures. The automation system is now operational and pleads for another decade of safe operations with XMM-Newton.

• May 2016: With 140 kg of propellant left onboard after the orbit injection maneuver, for a planned 10 years lifetime (elapsed in 2009) using approximately 6 kg propellant per year, propellant seemed not to be a life limiting factor for the mission, which scientific goal is to unveil the hot and violent universe with an unprecedented accuracy in terms of spectral and imaging detection capabilities. However the success of XMM-Newton reflected in the very high demand of observation time requested and the scientific output generated by the worldwide X-ray community turned this initially almost neglected factor into a major one, since the mission was extended various times because spacecraft and instruments are still operating without major degradation reducing the life limiting factors to the fuel reserve only. Measures to significantly reduce fuel consumption were already taken. Next, the Main Tank replenishing procedure had to be further developed, and in-flight tests were needed to determine performance and performance limits. Up to now, the RCS operated reliably, requiring only a minimum of attention. As long as there is enough propellant to keep all 4 tanks in wet conditions, the precise performance of the tank heater system, the accuracy of the 8 thermistors (2 on each tank), and the accurate knowledge of the gas temperature inside each tank were quite irrelevant issues. This is now radically changing with Main Tank depletion coming closer and with the need to develop, validate and implement the Main Tank replenishing procedure. 134)
- Because propellant migration and Main Tank replenishing can only be achieved by temperature changes, virtually all functions of the tank heater system and the thermal response of the individual tanks had to be tested and characterized by various in-flight tests. Taking into account the findings and experience gained by these in-flight tests and observing various constraints, a feasible Main Tank replenishing procedure was developed, which has enough performance margin to be called a “robust procedure”. The main parts of the procedure are already validated.
- Outlook: The large propellant gaging uncertainty is a very unsatisfactory. Attempts to reduce these uncertainties will continue in 2016. With the Main Tank replenishing procedure established, various mission planning and optimization exercises can be made, and a suitable planning tool to support operations later on should preserve the know-how gained.
• April 28, 2016: ESA's XMM-Newton has discovered gas streaming away at a quarter of the speed of light from very bright X-ray binaries in two nearby galaxies. At X-ray wavelengths, the celestial sky is dominated by two types of astronomical objects: supermassive black holes, sitting at the centers of large galaxies and ferociously devouring the material around them, and binary systems, consisting of a stellar remnant – a white dwarf, neutron star or black hole – feeding on gas from a companion star. 135) 136)
- In both cases, the gas forms a swirling disc around the compact and very dense central object: friction in the disc causes the gas to heat up and emit light at many wavelengths, with a peak in X-rays. Not all of the gas is swallowed by the central object though, and some of it might even be pushed away by powerful winds and jets.
- But an intermediate class of objects was discovered in the 1980s and is still not well understood. Ten to a hundred times brighter than ordinary X-ray binaries, these sources are nevertheless too faint to be linked to accreting supermassive black holes, and in any case, are usually found far from the center of their host galaxy. "We think these 'ultra-luminous X-ray sources' are somewhat special binary systems, sucking up gas at a much higher rate than an ordinary X-ray binary," explains Ciro Pinto from the Institute of Astronomy in Cambridge, UK. "Some host highly magnetized neutron stars, while others might conceal the long-sought-after intermediate-mass black holes, which have masses around 1000 times the mass of the Sun. But in the majority of cases, the reason for their extreme behavior is still unclear."
- Ciro and his colleagues delved into the XMM-Newton archives and collected several days' worth of observations of three ultra-luminous X-ray sources, all hosted in nearby galaxies located less than 22 million light-years from our Milky Way. The data were obtained over several years with the RGS (Reflection Grating Spectrometer), a highly sensitive instrument that allowed them to spot very subtle features in the spectrum of the X-rays from the sources.
- In all three sources, the scientists were able to identify X-ray emission from gas in the outer portions of the disc surrounding the central compact object, slowly flowing towards it. But two of the three sources – known as NGC 1313 X-1 and NGC 5408 X-1 – also show clear signs of X-rays being absorbed by gas that is streaming away from the central source at an extremely rapid 70 ,000 km/s – almost a quarter of the speed of light.
- "This is the first time we've seen winds streaming away from ultra-luminous X-ray sources," says Ciro. "And there's more, since the very high speed of these outflows is telling us something about the nature of the compact objects in these sources, which are frantically devouring matter."
- While the hot gas is pulled inwards by the central object's gravity, it also shines brightly, and the pressure exerted by the radiation pushes it outwards. This is a balancing act: the greater the mass, the faster it draws the surrounding gas. But this also causes the gas to heat up faster, emitting more light and increasing the pressure that blows the gas away.

Legend to Figure 108: Since gas cannot fall in from all directions in a rotating system, it forms a swirling disc around the compact object. This causes matter to heat up and emit light at many wavelengths, especially X-rays. However, not all the gas in the disc is swallowed, and some of it is blown away in the form of winds or jets. - Scientists using ESA's XMM-Newton have discovered gas streaming away at a quarter of the speed of light from two very bright X-ray binaries, known as ultra-luminous X-ray sources, that are located in nearby galaxies. The discovery confirms that these sources conceal a compact object accreting matter at extraordinarily high rates.
• March 31, 2016: Decades of searching in the Milky Way’s nearby ‘twin’ galaxy Andromeda have finally paid off, with the discovery of an elusive breed of stellar corpse, a neutron star, by ESA’s XMM-Newton space telescope. Andromeda, or M31, is a popular target among astronomers. Under clear, dark skies it is even visible to the naked eye. Its proximity and similarity in structure to our own spiral galaxy, the Milky Way, make it an important natural laboratory for astronomers. It has been extensively studied for decades by telescopes covering the whole electromagnetic spectrum. 137) 138)
- Despite being extremely well studied, one particular class of object had never been detected: spinning neutron stars. Neutron stars are the small and extraordinarily dense remains of a once-massive star that exploded as a powerful supernova at the end of its natural life. They often spin very rapidly and can sweep regular pulses of radiation towards Earth, like a lighthouse beacon appearing to flash on and off as it rotates.
- These ‘pulsars’ can be found in stellar couples, with the neutron star cannibalizing its neighbor. This can lead to the neutron star spinning faster, and to pulses of high-energy X-rays from hot gas being funnelled down magnetic fields on to the neutron star.
- Binary systems hosting a neutron star like this are quite common in our own Galaxy, but regular signals from such a pairing had never before been seen in Andromeda.
- Now, astronomers systematically searching through the archives of data from XMM-Newton X-ray telescope have uncovered the signal of an unusual source fitting the bill of a fast-spinning neutron star. It spins every 1.2 seconds, and appears to be feeding on a neighboring star that orbits it every 1.3 days.
- “We were expecting to detect periodic signals among the brightest X-ray objects in Andromeda, in line with what we already found during the 1960s and 1970s in our own Galaxy,” says Gian Luca Israel, from INAF-Osservatorio Astronomica di Roma, Italy, one of the authors of the paper describing the results, “But persistent, bright X-ray pulsars like this are still somewhat peculiar, so it was not completely a sure thing we would find one in Andromeda. “We looked through archival data of Andromeda spanning 2000–13, but it wasn’t until 2015 that we were finally able to identify this object in the galaxy’s outer spiral in just two of the 35 measurements.”
- While the precise nature of the system remains unclear, the data imply that it is unusual and exotic. “It could be what we call a ‘peculiar low-mass X-ray binary pulsar’ – in which the companion star is less massive than our Sun – or alternatively an intermediate-mass binary system, with a companion of about two solar masses,” says Paolo Esposito of INAF-Istituto di Astrofisica Spaziale e Fisica Cosmica, Milan, Italy.
- “We need to acquire more observations of the pulsar and its companion to help determine which scenario is more likely.”
- “The well-known Andromeda galaxy has long been a source of exciting discoveries, and now an intriguing periodic signal has been detected by our flagship X-ray mission,” adds Norbert Schartel, ESA’s XMM-Newton project scientist. “We’re in a better position now to uncover more objects like this in Andromeda, both with XMM-Newton and with future missions such as ESA’s next-generation high-energy observatory, Athena.”
![Figure 109: Andromeda, or M31, is a spiral galaxy similar to our own Milky Way. For the first time, a spinning neutron star has been inferred in XMM-Newton data [image credit: Andromeda: ESA/Herschel/PACS/SPIRE, J. Fritz, U. Gent, XMM-Newton/EPIC, W. Pietsch, MPE; data: P. Esposito et al., (2016)]](https://www.eoportal.org/ftp/satellite-missions/x/XMM-Newton_040722/XMM-Newton_Auto2B.jpeg)
Legend to Figure 109: Inset: Light curve of the source, known as 3XMM J004301.4+413017, as analyzed by XMM-Newton’s European Photon Imaging Camera, EPIC. The source has a period of 1.2 seconds, consistent with a spinning neutron star.
• December 15, 2015: Today marks the release of the first papers to result from the XXL survey, the largest survey of galaxy clusters ever undertaken with ESA's XMM-Newton X-ray observatory. The gargantuan clusters of galaxies surveyed are key features of the large-scale structure of the Universe and to better understand them is to better understand this structure and the circumstances that led to its evolution. The first results from the survey, published in a special issue of Astronomy and Astrophysics, hint at the answers and surprises that are captured in this unique bank of data and reveal the true potential of the survey. 139)
- The XXL project, the largest XMM-Newton observing program to date, set itself the ambitious task of mapping galaxy clusters back to a time when the Universe was just half of its present age. Its aim was to trace the evolution of the large-scale structure of the Universe. When looking at the large-scale distribution of matter in the Universe we see that it is not evenly distributed throughout space but forms a cosmic web of matter-filled filaments and a network of vast matter-less voids. Within these filaments huge swathes of matter have been pulled together by the effect of gravity into galaxy clusters, the largest bound entities in the Universe. Studying how these clusters evolve over time, in surveys like XXL, holds the key to uncovering how the arrangement of matter in the Universe has changed – the dense getting denser and the voids becoming emptier – and gives valuable insights into the accelerated expansion of the Universe and the factors that drive it.
- "Tracing the evolution of cosmic structure is of special interest to astronomers," explains Marguerite Pierre, principal investigator of the XXL project. "How the structure has evolved depends on certain important factors like the density of the Universe and the acceleration of its expansion. The better our knowledge of how this structure has evolved, the more accurate our models of the Universe become. This in turn helps us to dig deeper into the nature of the most mysterious components of our Universe – dark matter and dark energy."
- The XXL survey covered two huge regions each of which, if viewed on the sky, would have a two-dimensional area a hundred times larger than the full Moon, and that is without taking into account the depth that the survey explored. These already extensive swathes of sky were mapped back to a time when the Universe was half of its present age with a total of 450 galaxy clusters detected, and their properties and distributions mapped, in the process. This is no mean feat as, for many clusters, fewer X-ray photons were detected per cluster than there are galaxies in that cluster. Despite this meager amount of information the team was still able to identify clusters much more easily than would be possible in visible or infrared light by detecting the huge reservoirs of gas that fill the space between the resident galaxies, rather than trying to pick out the light emitted by the visible matter in the clusters. These gas reservoirs are heated to a few tens of millions of degrees and can be detected as X-ray emission.
- Today, the XXL team present their first results in a set of thirteen papers published in a special issue of Astronomy and Astrophysics. Among these initial findings, which are based on a catalog of the 100 brightest clusters in the survey – also released today – the promise of shedding new light on dark energy and matter is already being met, with evidence emerging that could lead to refinements of current cosmological models. 140)
- Prior to the survey the XXL team made predictions about the number of clusters they expected to find, and how densely they expected them to be spread across the sky, by using data from ESA's Planck telescope, which measures the remnant radiation from the Big Bang. To do this requires careful calculation of how the Universe has expanded since its inception, a phenomenon driven by the energy content of the Universe. When the team analyzed the data from XXL they found that the density of clusters was in fact lower than had been predicted by modelling using Planck data. Although the reason for the discrepancy remains unknown, it could imply that our Universe is somewhat different or more complex than the current cosmological model favored by Planck or, alternatively, that our understanding of the physics and evolution of galaxy clusters needs to be improved. Further investigation will be carried out with the full sample of clusters in 2017.

Legend to Figure 110: XXL is the largest survey of galaxy clusters ever undertaken and provides by far the best view of the deep X-ray sky yet obtained. The survey was carried out with ESA's XMM-Newton X-ray observatory. The area shown in this image was obtained with some 220 XMM-Newton pointings and, if viewed on the sky, would have a two-dimensional area a hundred times larger than the full Moon (which spans one half degree), and that is without taking into account the depth that the survey explores. The red circles in this image show the clusters of galaxies detected in the survey. Along with the other field – XXL-North field (or XXL-N) – around 450 of these clusters were uncovered in the survey, which mapped them back to a time when the Universe was just half of its present age. The image also reveals some of the 12 000 Active Galactic Nuclei that were detected in the field.
• December 3, 2015: Scientists have developed a technique to use quasars – powerful sources driven by supermassive black holes at the center of galaxies – to study the Universe's history and composition. To demonstrate the new method, based on a relation between a quasar's luminosity at X-ray and ultraviolet wavelengths, they made extensive use of data from ESA's XMM-Newton X-ray observatory. This approach promises to become an important tool to constrain the properties of our Universe. 141)
- At the core of most massive galaxies in the Universe is a supermassive black hole – a concentration of matter so dense that it attracts anything nearby, including light. Such black holes have masses from millions to billions of times that of the Sun and are generally idle, only accreting the occasional star or gas cloud that ventures too close to the galaxy's center.
- A small fraction of them are, however, extremely active, devouring matter at a very high rate, causing the surrounding material to shine brightly across the electromagnetic spectrum, from radio waves to X-rays and gamma rays. In some cases, emission from matter in the vicinity of the black hole is so intense that the core of the galaxy outshines the stars. These objects appear as point sources in the sky, like stars, and are known as quasars – short for quasi-stellar sources.
- Quasars allow scientists to study gravity in the very strong field of the supermassive black holes. In addition, comparing the properties of quasars with those of other galaxies that host either active or passive black holes can reveal interesting aspects about the evolution of galaxies over cosmic history.
- But one other aspect piqued the interest of two scientists from the Arcetri Astrophysical Observatory in Firenze, Italy: they realized that quasars can be used as probes of the expansion history of the Universe. 142)

Legend to Figure 111: Actively accreting supermassive black holes devour matter at very high rate, causing the surrounding material to shine brightly across the electromagnetic spectrum, from radio waves to X-rays and gamma rays. In some cases, emission from matter in the vicinity of the black hole is so intense that the core of the galaxy outshines the total luminosity of its stars: as a result, these objects appear as point sources in the sky, like stars, and are known as quasars – short for quasi-stellar sources.
As the accreted material flows towards the black hole through a disc, it is heated by friction and shines brightly at visible and ultraviolet wavelengths, shown here in red and yellow, respectively. Part of the light emitted by the disc interacts with highly energetic electrons in a corona near the disc (shown in blue), receiving an extra energy boost and turning into X-rays.
• August 20, 2015: This new image of powerful remnants of dead stars and their mighty action on the surrounding gas from ESA's XMM-Newton X-ray observatory reveals some of the most intense processes taking place at the center of our galaxy, the Milky Way (Figure 112). The bright, point-like sources that stand out across the image trace binary stellar systems in which one of the stars has reached the end of its life, evolving into a compact and dense object – a neutron star or black hole. Because of their high densities, these compact remnants devour mass from their companion star, heating the material up and causing it to shine brightly in X-rays. 143) 144)
- The central region of our galaxy also contains young stars and stellar clusters, and some of these are visible as white or red sources sprinkled throughout the image, which spans about one thousand light-years. Most of the action is occurring at the center, where diffuse clouds of gas are being carved by powerful winds blown by young stars, as well as by supernovas, the explosive demise of massive stars.
- The supermassive black hole sitting at the center of the Galaxy is also responsible for some of this action. Known as Sagittarius A*, this black hole has a mass a few million times that of our Sun, and it is located within the bright, fuzzy source to the right of the image center.
- While black holes themselves do not emit light, their immense gravitational pull draws in the surrounding matter that, in the process, emits light at many wavelengths, most notably X-rays. In addition, two lobes of hot gas can be seen extending above and below the black hole.
- Astronomers believe that these lobes are caused either directly by the black hole, which swallows part of the material that flows onto it but spews out most of it, or by the cumulative effect of the numerous stellar winds and supernova explosions that occur in such a dense environment.

Legend to Figure 112: This image portrays powerful remnants of dead stars and their mighty action on the surrounding gas, showing us an unprecedented view of the Milky Way's energetic core. It was put together in a new study by compiling all observations of this region that were performed with XMM-Newton, adding up to over one month of monitoring in total. — The image combines data collected at energies from 0.5 to 2 keV (shown in red), 2 to 4.5 keV (shown in green) and 4.5 to 12 keV (shown in blue). It spans about 2.5º across, equivalent to about one thousand light-years. — This image, showing us an unprecedented view of the Milky Way's energetic core, was put together in a new study by compiling all observations of this region that were performed with XMM-Newton, adding up to about one and a half months of monitoring in total.
• February 19, 2015: Astronomers have discovered that the winds from supermassive black holes at the center of galaxies are blasted out in all directions. This new finding was made possible by observations with ESA's XMM-Newton and NASA's NuSTAR X-ray telescopes and it supports the picture of black holes having a significant impact on star formation of their host galaxy. 145) 146)
- At the core of every massive galaxy in the Universe sits a supermassive black hole, with a mass some millions or billions of times that of our Sun. Some of these black holes are active, meaning that there is a frenzied flow of matter in their vicinity: the intense gravitational pull of the black hole causes matter to spiral towards it in an accretion disc and at the same time part of that matter is cast away through powerful winds.
- For the past few decades, astronomers have investigated if and how these inflows and outflows of matter may have large-scale effects on the host galaxy and, in particular, on its star formation activity. While such an interaction would explain several observations, including the correlation between the mass of stars in the bulge of a galaxy and the mass of its central black hole, it is by no means obvious that the black hole could have an impact on its host galaxy as a whole.
- "Black holes are powerful objects, but their gravitational influence does not extend much beyond the very inner parts of a galaxy," explains Emanuele Nardini of Keele University, UK. "If black holes are really to influence the star-forming activity of an entire galaxy, there must be a feedback mechanism connecting the two on a global scale." One possibility is that the winds driven by the black hole's accretion activity play a role and, as reported in the journal Science, Nardini and collaborators have obtained the first solid evidence supporting this scenario.
- The team have looked at PDS 456, a galaxy that lies just over two billion light-years away and that hosts an exceptionally active black hole with a mass of one billion Suns. PDS 456 is a quasar, a class of galaxies that appear as a point source because the brightness produced by their stars is outshone by the emission from the activity of the central black hole.
- Due to the high temperatures caused by friction in the material flowing towards the black hole, the accreted material shines brightly at optical and ultraviolet wavelengths, and subsequent interactions of this light with highly energetic electrons in the immediate surroundings of the disc also produce copious amounts of X-rays. A comprehensive view of the accreting activity of this quasar could be obtained by observing PDS 456 simultaneously with ESA's XMM-Newton X-ray observatory and NASA's NuSTAR (Nuclear Spectroscopic Telescope Array ) mission. The observations were performed on four occasions in 2013, and once again in 2014.

Legend to Figure 113: A study based on joint observations with ESA's XMM-Newton and NASA's NuSTAR X-ray telescopes of the quasar PDS 456, which hosts a very active black hole, has shown that the winds driven by a black hole can be wide and almost spherical. This discovery supports the picture of black holes having a significant impact on star formation of their host galaxy.
• December 19, 2014: First impressions can be deceptive – astronomers have used ESA's X-ray satellite XMM-Newton to find a massive black hole hungrily feeding within a tiny dwarf galaxy (Figure 114), despite there being no hint of this black hole from optical observations. 147) 148)
- The galaxy, an irregular dwarf named J1329+3234, is one of the smallest galaxies yet to contain evidence of a massive black hole. Located over 200 million light-years away, the galaxy is similar in size to the Small Magellanic Cloud, one of our nearest neighboring galaxies, and contains a few hundred million stars.
- In 2013, an international team of astronomers was intrigued to discover infrared signatures of an accreting black hole within J1329+3234 when they studied it with the Wide-Field Infrared Survey Explorer (WISE).
- The same team has now investigated the galaxy further, using ESA's XMM-Newton to hunt for this black hole in X-rays – and found something very surprising.
- "The X-ray emission from J1329+3234 is over 100 times stronger than expected for this galaxy," says Nathan Secrest of George Mason University in Virginia, USA, lead author of the new study published in The Astrophysical Journal. "We would typically expect to find low-level X-ray emission from stellar-mass black holes within the galaxy, but what we found instead was emission consistent with a very massive black hole."
- The combined X-ray and infrared properties of this galaxy can only be explained by the presence of a massive black hole residing in J1329+3234, similar to the supermassive black holes found at the centers of much more massive galaxies.
- While the exact mass of the black hole is not known, it must be at least 3000 times as massive as the Sun, although it is likely to be much more massive than that. If the black hole in J1329+3234 is similar to known low-mass supermassive black holes, then it has a mass of around 150, 000 times that of the Sun.
- A feeding black hole at the center of a galaxy is known as an AGN (Active Galactic Nucleus). In the region surrounding the black hole, material from the galaxy emits intensely bright radiation as it swirls inwards towards the center of the galaxy and is devoured by the black hole. AGNs powered by massive black holes are commonplace in large galaxies, but they appear to be rarer in galaxies without a central "bulge" of stars – dwarf galaxies being a key example.
- "This is a really important discovery," says co-author Shobita Satyapal, also from George Mason University. "It's interesting enough that such a tiny galaxy has such a large black hole, but this also raises questions about how these black holes form in the first place."
- Astronomers believe that the "seeds" of massive black holes formed very early on in the Universe, along with the first generation of stars. These seed black holes then grew into massive black holes via a string of galaxy mergers. As the galaxies merged, so did their central black holes.
![Figure 114: This image depicts the X-ray emission from dwarf galaxy J1329+3234 (center in this image), and from a background AGN (lower right), measured by XMM-Newton in June 2013 [image credit: SA/XMM-Newton, N. Secrest, et al. (2015)]](https://www.eoportal.org/ftp/satellite-missions/x/XMM-Newton_040722/XMM-Newton_Auto26.jpeg)
Legend to Figure 114: The image is constructed from 2-10 keV X-ray emission and has been smoothed. The color code represents the intensity of X-ray emission with blue being more intense and red less intense. The white bar indicates a width of 10 arcseconds, equivalent to 3.3 kpc at the distance of this galaxy. North is up, east to the left.
• December 15, 2015: Today marks the release of the first papers to result from the XXL survey, the largest survey of galaxy clusters ever undertaken with ESA's XMM-Newton X-ray observatory. The gargantuan clusters of galaxies surveyed are key features of the large-scale structure of the Universe and to better understand them is to better understand this structure and the circumstances that led to its evolution. The first results from the survey, published in a special issue of Astronomy and Astrophysics, hint at the answers and surprises that are captured in this unique bank of data and reveal the true potential of the survey. 149)
- The XXL project, the largest XMM-Newton observing program to date, set itself the ambitious task of mapping galaxy clusters back to a time when the Universe was just half of its present age. Its aim was to trace the evolution of the large-scale structure of the Universe.
• November 20, 2014: Mission extension for XMM-Newtom. During its meeting at ESAC (European Space Astronomy Center), near Madrid, on 19 November, the SPC (Science Program Committee) gave the green light for the flotilla of spacecraft to continue their key scientific endeavors for at least another two years. 150)
- After a comprehensive review by the Science Program’s advisory structure of the current operational status and likely scientific return of each mission in the future, the SPC agreed to continue funding for six ESA-led missions (Cluster, INTEGRAL, Mars Express, PROBA-2, SOHO and XMM-Newton) for the period 1 January 2015 – 31 December 2016.
- XMM-Newton, the Hubble Space Telescope and INTEGRAL will continue to provide complementary, multi-wavelength observations of the Universe. These will span studies of the Solar System, planet-hosting stars, exploding stars, black holes, and the evolution of galaxies.
• July 11, 2014: ESA’s XMM-Newton observatory has helped to uncover how the Universe’s first stars ended their lives in giant explosions. Astronomers studied the gamma-ray burst GRB130925A – a flash of very energetic radiation streaming from a star in a distant galaxy 5.6 billion light years from Earth – using space- and ground-based observatories. They found the culprit producing the burst to be a massive star, known as a blue supergiant. These huge stars are quite rare in the relatively nearby Universe where GRB130925A is located, but are thought to have been very common in the early Universe, with almost all of the very first stars having evolved into them over the course of their short lives. 151) 152)
- But unlike other blue supergiants we see nearby, GRB130925A's progenitor star contained very little in the way of elements heavier than hydrogen and helium. The same was true for the first stars to form in the Universe, making GRB130925A a remarkable analogue for similar explosions that occurred just a few hundred million years after the Big Bang.

Legend to Figure 115: Astronomers used a number of space- and ground-based observatories, including ESA's XMM-Newton to study the gamma-ray burst GRB130925A – a flash of very energetic radiation streaming from a blue supergiant in a galaxy 5.6 billion light years from Earth. They found evidence that this star contained very little in the way of elements heavier than hydrogen and helium. The same was true for the first stars to form in the Universe, making GRB130925A a remarkable analog for similar explosions that occurred just a few hundred million years after the Big Bang.
• General status of the XMM-Newton spacecraft as of May 2014: 153)
All AOCS units are currently operating on the prime branch and do not show any significant sign of degradation. The complete AOCS redundant units are available and untouched for nominal operations. Relevant ON/OFF cycles and life limited parts of Inertia Measurement Units (IMU), Reaction Wheels (RW), Flow Control Valves (FCV), Catalyst Bed Heaters (CBH), and Latch Valves (LV) are well below critical margins. Performances of all the subunits (e.g. thrusters and reaction wheels) do not show any unexpected major degradation, where two of the reaction wheels show indication of increased bearing noise which however has been reduced by various counter measures . Thrusters degradation at current degradation rate is estimated to be 10% during the extended lifetime by manufacturer. The A unit of the star tracker suffers from some blemish pixels. Right now operations that would require the use of those pixels are avoided with a ground based filtering by the Flight Dynamics system. However a new approach using the on board offset table for the star tracker to correct for the wrong behavior of hot pixels is under investigation for implementation if the number of hot pixels would get to high.
- Automation on board: The XMM-Newton platform design is to fail safe after any single anomaly and remain at a safe attitude until recovery is possible from ground command. In case of loss of contact, the mission operations concept is designed to ensure both platform and instruments remain safe for at least 36 hours. The CDMU “autonomy” functions are limited to a time-tagged command buffer and a number of mission specific monitoring and control tasks. The timetagged command buffer is used to: ensure instruments are commanded safe before perigee passage; maintain instrument thermal control during eclipse; and since a platform thermistor failure in 2009 to maintain tank heater control. A monitor and control task to command instruments safe in case of ESAM was provided in the launch version of the CDMU software. A new task was uplinked in 2001 to monitor and control the prime instrument CCD temperature. In 2014 it is planned to uplink and commission two further tasks: a thermal duty cycle (TDC) function and a thermal closed loop (TCL) function. The former allows parallel control of a number of heater circuits by commanding the heater transistor switch to a predefined duty cycle. This will be used primarily for fine control of heater power input needed for each tank replenishing event. The latter allows closed loop control of a number of heater circuits by monitoring the associated thermistor temperature and commanding the heater switch to maintain a limit cycle between minimum and maximum values. This will be used both for tank temperature control in between tank replenishing events and for autonomous instrument heater control during and after eclipses affected by long ground station gaps.
- Automation on ground: The original design of XMM-Newton operations was based on a continuous ground station coverage and cannot be changed anymore, since the spacecraft has no mass memory on board. Telecommanding and Telemetry reception is therefore handled “live” from the MCS (Mission Control System) with individual commands, which are however for routine operations prepared and bundled into an automated timeline. The timeline runs in real time and sends all the required commands. Verification of telecommanding is done via the SCOS 2000 system through the SPACON (SPAcecraft CONtroller) supported by out-off-limits warnings from SCOS 2000. Additional commands or recovery actions have to be send manually by the SPACON using flight operation procedures. In order to support the SPACON in his activities a system is currently been developed to automate some of these procedures using a platform called MOIS (Manufacturing and Operations Information System).
Offline, using the so called MOIS Scheduler, a plan is generated for each revolution and contains the orbit constraints, the ground station schedule and the science activities. The automated version of a traditional flight operation procedure is then linked to a generic event of the timeline and executes at runtime. Furthermore the MOIS Executor is able to read telemetry and react to it by sending telecommand and interacting with the mission control system. Repeating operations are hence triggered automatically and will reduce human intervention. Automation is especially useful to execute routine operations which are not part of the timeline such as eclipse operations or calibration activities. Moreover, MOIS has been set up to cope with telemetry out-of-limit. In case of anomaly, the mission control system raises a warning flag and notifies the automation system. The MOIS Event Monitor initiates then the execution of the recovery procedure. The operational philosophy for introducing the automation on ground is to start with nominal operations and eventually after gaining experience adding as well contingency operations.

Fuel | remaining | 55 kg |
Solar array power | Maximum required | 1350 W |
Gyros | Usage | < 21 % |
Reaction wheels | Usage | < 40 % |
Rf switches
| Usage | Stuck at one position. Back up not used instead transponders are switched |

- In summary, ESA’s XMM-Newton X-ray space observatory is after nearly 15 years in orbit operating without any significant unexpected degradation. The only life limiting item being the hydrazine reserves on board has been extended by various measures from 2019 to 2027 with a potential improvement to 2030 currently under investigation. The main factor for fuel saving was a change to the on-board software of the Attitude Control Computer to allow operating all four reaction wheels in parallel instead of only running three of them as done previously. This method offered as well the possibility to apply measures against increased bearing noise, which has been detected on two of the XMM-Newton reaction wheels. We have been able to reduce the bearing noise fraction from ~40 % to less than 8 % for RW1 and below the detection threshold for RW2. Given this highly encouraging perspective the mission is being prepared for a potential future of up to 15 years, developing plans to operate the Hydrazine Propulsion System in the so-called near fuel depletion regime at the end of the technical life limit. The ground segment hardware has been migrated to state of the art generic virtualized systems with higher performance and greater redundancy. Furthermore ground and on board automation possibilities are being developed to make operations robust and more efficient.
• April 22, 2014: A pair of supermassive black holes in orbit around one another have been spotted by XMM-Newton. This is the first time such a pair have been seen in an ordinary galaxy. They were discovered because they ripped apart a star when the space observatory happened to be looking in their direction. 154) 155)
- Most massive galaxies in the Universe are thought to harbor at least one supermassive black hole at their center. Two supermassive black holes are the smoking gun that the galaxy has merged with another. Thus, finding binary supermassive black holes can tell astronomers about how galaxies evolved into their present-day shapes and sizes.
- To date, only a few candidates for close binary supermassive black holes have been found. All are in active galaxies where they are constantly ripping gas clouds apart, in the prelude to crushing them out of existence.
- In the process of destruction, the gas is heated so much that it shines at many wavelengths, including X-rays. This gives the galaxy an unusually bright center, and leads to it being called active. The new discovery, reported by Fukun Liu, Peking University, Beijing, China, and colleagues, is important because it is the first to be found in a galaxy that is not active.

• March 5, 2014: Astronomers have used NASA's Chandra X-ray Observatory and the ESA's XMM-Newton to show a supermassive black hole six billion light years from Earth is spinning extremely rapidly. This first direct measurement of the spin of such a distant black hole is an important advance for understanding how black holes grow over time. 156)
- Multiple images of a distant quasar are visible in this combined view from NASA's Chandra X-ray Observatory and the Hubble Space Telescope. The Chandra data, along with data from ESA's XMM-Newton, were used to directly measure the spin of the supermassive black hole powering this quasar. This is the most distant black hole where such a measurement has been made.
- Reis and his colleagues determined the spin of the supermassive black hole that is pulling in surrounding gas, producing an extremely luminous quasar known as RX J1131-1231 (RX J1131 for short). Because of fortuitous alignment, the distortion of space-time by the gravitational field of a giant elliptical galaxy along the line of sight to the quasar acts as a gravitational lens that magnifies the light from the quasar. Gravitational lensing, first predicted by Einstein, offers a rare opportunity to study the innermost region in distant quasars by acting as a natural telescope and magnifying the light from these sources.

• November 13, 2013: Astronomers studying a black hole in our Galaxy with ESA’s XMM-Newton observatory have made a surprising discovery about the cocktail of particles that are ejected from its surroundings. Stellar-mass black holes are often found feasting on material from a companion star. Matter flows from the star towards the black hole, circling in a disc around it with a temperature so high that it emits X-rays. 157) 158)
- The black hole can be a fussy eater: instead of swallowing all of the material, it sometimes pushes a fraction of it away in the form of two powerful jets of particles. Because these jets release mass and energy into the surroundings, the black hole has less material to feed on. By studying the composition of the jets, we can learn more about the feeding habits of black holes.
- Observations at radio wavelengths have already found that black hole jets contain electrons moving at close to the speed of light. But, until now, it was not clear whether the negative charge of the electrons is complemented by their anti-particles, positrons, or rather by heavier positively-charged particles in the jets, like protons or atomic nuclei.
- In a new study, astronomers have used XMM-Newton to study a black hole binary system called 4U1630–47, well known to show outbursts of X-rays over periods of months and years.
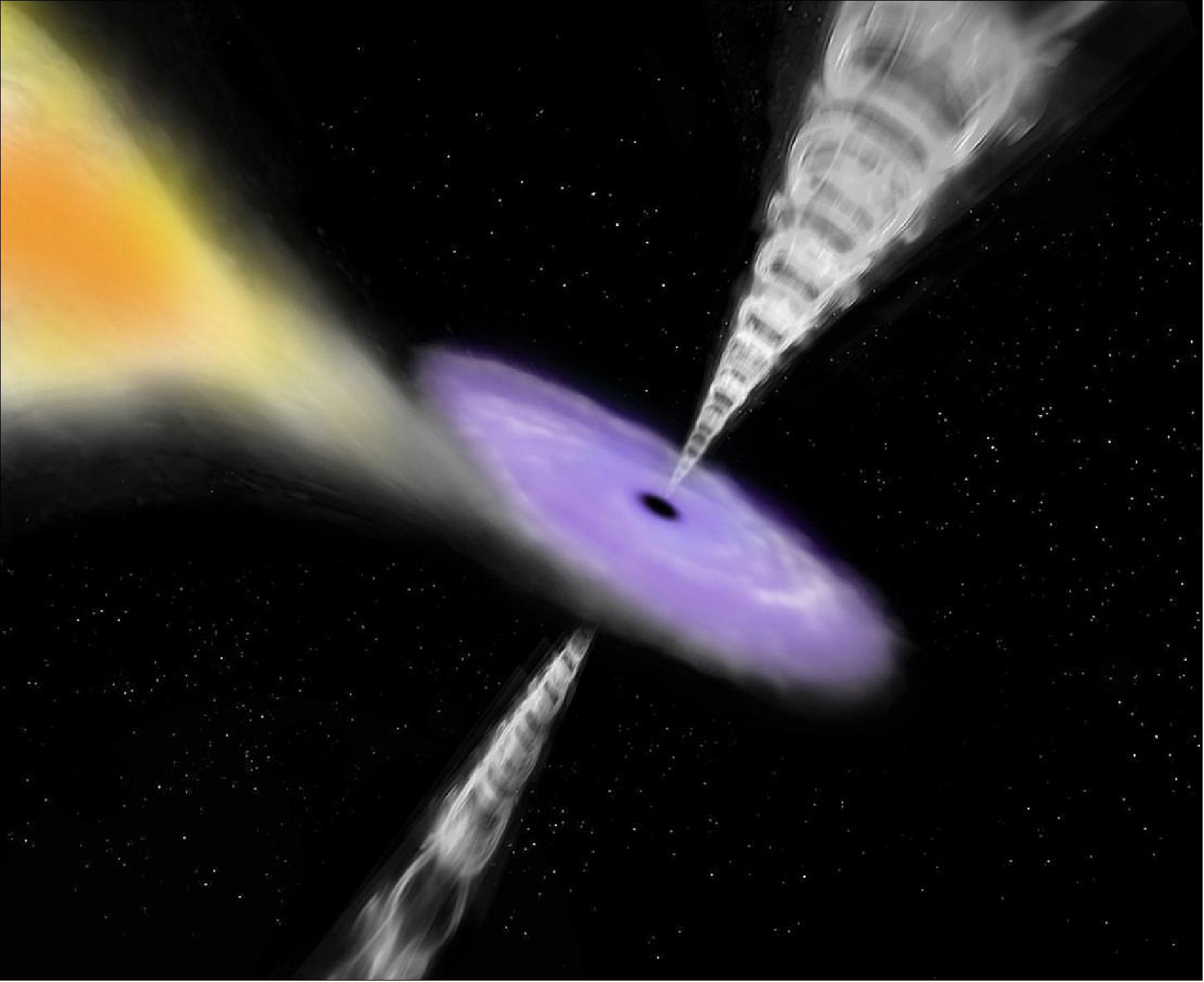
Legend to Figure 120: A team of astronomers studying the jets of the binary system 4U1630-47 have confirmed that black hole jets not only consist of electrons but also contain heavier particles, like protons or atomic nuclei. This means that jets can carry mass and energy away from the black hole in much larger amounts than previously thought.
• In July 2013, ESA released a new catalog from the XMM-Newton space telescope which provides an unprecedented cosmic X-ray library for the exploration of the extreme Universe. The third XMM-Newton Serendipitous Source Catalog (3XMM-DR4) contains more than half a million sources, all of which are provided to a better quality than ever before. Improved data processing means that source identification is more reliable, and fainter objects are detected. 159)
- The catalogue provides an exceptional dataset for generating large, well-defined samples of objects such as active galaxies (which dominate the detections in this catalogue), clusters of galaxies, interacting compact binaries, and active stellar coronae. This vast inventory is also home to some of the rarest and most extreme phenomena in the Universe, such as tidal disruption events - when a black hole swallows another star, producing prodigious outbursts of X-ray emission.
- "The catalogue provides plenty of scope for new discoveries as well as in-depth studies of large samples," says Professor Mike Watson of the University of Leicester, who leads the XMM-Newton Survey Science Center (SSC). "XMM-Newton is pre-eminent amongst current X-ray missions in its ability to perform 'survey' science, with a chance to find previously undetected objects and then explore their properties."
- The sources in the 3XMM catalogue are identified and isolated from serendipitous data recorded by XMM-Newton's EPIC X-ray cameras. In each of the 600-700 observations made each year, around 70 extra sources are captured in addition to the target object which usually only takes up a small fraction of the field of view. Covering observations between February 2000 and December 2012, the catalogue contains some 531 261 X-ray source detections relating to 372 728 unique X-ray sources.
- "The third XMM-Newton Serendipitous Source Catalogue shows how much added value can be gained from the observations," notes Watson. "I'd like to pay tribute to the efforts of the whole team which were crucial to completing this major undertaking."
- Natalie Webb of the Institut de Recherche en Astrophysique et Planétologie, Toulouse is taking over from Leicester's Simon Rosen as the SSC Manager for the next phase of the project. She comments: "Previous versions of the catalogue have yielded unexpected and exciting results, and with around 50 per cent more data now available, there should be plenty more to come. I look forward to the SSC continuing to play its leading role in this area."
- Coinciding with the publication of the 3XMM catalogue, a new version of the XMM-Newton Science Archive (XSA) is released, providing convenient access to the catalogue. The most visible change in XSA is the new web-based interface, but behind the scenes many changes have been introduced to make it easier and quicker to find and use the data.
- The 3XMM-DR4 catalogue is the sixth publicly released XMM-Newton X-ray source catalogue produced by the XMM-Newton Survey Science Center consortium on behalf of ESA.
- The XMM-Newton Survey Science Center, led by Professor Mike Watson at the University of Leicester, is a consortium of the following institutions:
University of Leicester, United Kingdom
Mullard Space Science Laboratory, University College London, United Kingdom
Institute of Astronomy, Cambridge, United Kingdom
Max-Planck Institut für extraterrestrische Physik, Garching, Germany
Astrophysikalisches Institut, Potsdam, Germany
Service d'Astrophysique, CEA/DSM/Dapnia, Saclay, France
Institut de Recherche en Astrophysique et Planétologie, Toulouse, France
Observatoire Astronomique de Strasbourg, France
Instituto de Fisica de Cantabria, Santander, Spain
Osservatorio Astronomico di Brera, Milan, Italy.

Legend to Figure 121: The sources in the 3XMM catalog are identified and isolated from serendipitous data recorded by XMM-Newton's EPIC X-ray cameras. In each of the 600-700 observations made each year, around 70 extra sources are captured in addition to the target object which usually only takes up a small fraction of the field of view. Covering observations between February 2000 and December 2012, the catalog contains some 531 261 X-ray source detections relating to 372 728 unique X-ray sources. — The inset shows an expanded view of the small region indicated where the individual sources can be seen.
• June 4, 2013: The XMM-Newton mission is in operation; it is detecting more X-ray sources than any previous satellite and is helping to solve many cosmic mysteries of the violent Universe, from what happens in and around black holes to the formation of galaxies in the early Universe. It is designed and built to return data for at least a decade. It is the biggest science satellite ever built in Europe. Its telescope mirrors are the most sensitive ever developed in the world, and with its sensitive detectors, it sees much more than any previous X-ray satellite. 160)
- XMM-Newton has been able to measure for the first time the influence of the gravitational field of a neutron star on the light it emits. This measurement provides much better insight into these objects. Neutron stars are among the densest objects in the Universe — a sugarcube-sized piece of a neutron star would weigh over a thousand million tonnes. Neutron stars are the remnants of heavy stars that end their life in a supernova explosion. In such cataclysmic events, most of the stellar matter is ejected into space (to eventually become the building blocks of all matter in the Universe, including ourselves). Part of what remains then collapses under its own gravity.
- Scientists believe that, in a neutron star, the density and the temperatures are similar to those existing a fraction of a second after the Big Bang when the primordial soup of matter in the Universe was ‘broken’ into its most fundamental constituents. They assume that when matter is tightly packed as it is in a neutron star, it goes through important changes. Protons, electrons, and neutrons — the components of atoms — fuse together. It is possible that even the building blocks of protons and neutrons, the so-called quarks, get crushed together.
- Scientists have spent the last decades trying to identify the nature of matter in neutron stars. To do this, they need to know some important parameters, very precisely. If you know a star’s mass and radius, or the relationship between them, you can obtain its density. However, no instrument was advanced enough to perform the measurements needed, until now. Thanks to ESA’s XMM-Newton observatory, astronomers have been able to obtain the mass-to-radius ratio of a neutron star for the first time and acquire the first clues about its composition. These clues suggest that neutron stars contain normal, non-exotic matter, although they are not conclusive. Scientists say this is a ‘key first step’ and that they will keep on with the search.
- This measurement was a first in astronomical observation and it is considered a huge achievement. The method consists of determining the compactness of the neutron star in an indirect way. The gravitational pull of a neutron star is immense — thousands of million times stronger than the Earth’s. This makes the light emitted by the neutron star lose energy. This energy loss is called a gravitational ‘redshift’. The measurement of this redshift by XMM-Newton indicated the strength of the gravitational pull, and revealed the star’s compactness.
• February 27, 2013: A rapidly rotating supermassive black hole has been found in the heart of a spiral galaxy by ESA’s XMM-Newton and NASA’s NuSTAR space observatories, opening a new window into how galaxies grow. Supermassive black holes are thought to lurk in the center of almost all large galaxies, and scientists believe that the evolution of a galaxy is inextricably linked with the evolution of its black hole. 161) 162)
- How fast a black hole spins is thought to reflect the history of its formation. In this picture, a black hole that grows steadily, fed by a uniform flow of matter spiralling in, should end up spinning rapidly. Rapid rotation could also be the result of two smaller black holes merging.

Legend to Figure 122: Depicted in this image is an outflowing jet of energetic particles, believed to be powered by the black hole’s spin. The regions near black holes contain compact sources of high energy X-ray radiation thought, in some scenarios, to originate from the base of these jets. The nature of the X-ray emission enables astronomers to see how fast matter is swirling in the inner region of the disc, and ultimately to measure the black hole's spin rate.
• January 24, 2013: New observations of a highly variable pulsar using ESA's XMM-Newton are perplexing astronomers. Monitoring this pulsar simultaneously in X-rays and radio waves, astronomers have revealed that this source, whose radio emission is known to 'switch on and off' periodically, exhibits the same behavior, but in reverse, when observed at X-ray wavelengths. It is the first time that a switching X-ray emission has been detected from a pulsar, and the properties of this emission are unexpectedly puzzling. As no current model is able to explain this switching behavior, which occurs within only a few seconds, these observations have reopened the debate about the physical mechanisms powering the emission from pulsars. 164) 165)
- Few classes of astronomical objects are as baffling as pulsars – which were discovered as flickering sources of radio waves and soon after interpreted as rapidly rotating and strongly magnetized neutron stars. Even though about 2000 pulsars have been found since the first was discovered in 1967, a detailed understanding of the mechanisms that power them still eludes astronomers.
- "There is a general agreement about the origin of the radio emission from pulsars: it is caused by highly energetic electrons, positrons and ions moving along the field lines of the pulsar's magnetic field, and we see it pulsate because the rotation and magnetic axes are misaligned," explains Wim Hermsen from SRON, the Netherlands Institute for Space Research in Utrecht, The Netherlands. "How exactly the particles are stripped off the neutron star's surface and accelerated to such high energy, however, is still largely unclear," he adds.
- "Many pulsars have a rather erratic behavior: in the space of a few seconds, their emission becomes weaker or even disappears for a while, just to go back to the previous level after some hours," says Hermsen. "We do not know what causes such a switch, but the fact that the pulsar keeps memory of its previous state and goes back to it suggests that it must be something fundamental."
- Recent studies indicate that the switch between what are usually referred to as 'radio-bright' and 'radio-quiet' states is correlated to the pulsar's dynamics. As pulsars rotate, their spinning period slows down gradually, and in some cases the slow-down process has been observed to accelerate and slow down again, in conjunction with the pulsar switching between radio-bright and quiet states. The existence of correlated variations in both the rotation and emission suggest a connection between a pulsar's immediate vicinity and, on a grander scale, its co-rotating magnetosphere, which may extend up to about 50 000 km for objects like PSR B0943+10. In order for the radio emission to vary so radically on the short timescales observed, the pulsar's global environment must undergo a very rapid – and reversible – transformation.

• December 11, 2012: At about 06:51 hrs UT on 11 December 2012, during XMM-Newton revolution 2382, an event was registered in the focal plane of the EPIC MOS1 instrument. The characteristics of the event were reminiscent of a similar event registered in the MOS1 focal plane on 9 March 2005 and of other less energetic events registered in the MOS1 focal plane on 17 September 2001, the MOS2 focal plane on 12 August 2002, and the pn focal plane on 19 October 2000, which were attributed to micrometeoroid impacts scattering debris into the focal plane. In each MOS case a bright flash of light caused data buffer overflows for the CCDs across the whole focal plane, and in all cases a number of new hot or defective pixels were subsequently mapped and masked. The consequences of the event on 9 March 2005 were more significant meaning, in addition, the loss of one of the seven MOS1 CCD detectors, namely CCD6: 166)
- After the event on 11 December 2012, another peripheral CCD, namely MOS1 CCD3, is significantly damaged.
- No impact on the other X-ray instruments has been observed.
- Scientific observations are continuing normally with XMM-Newton, including MOS1, but now without data from CCD3, or from CCD6 as a result of the 2005 event.
- The science impact of the loss of MOS1 CCD3 is small.
CCD3 is one out of the six original peripheral CCDs of MOS1. It covers, to a first order approximation, slightly less than 1/7 (or 14%) of the geometrical area of MOS1. MOS1, in turn, only contains some 22% of the total effective area of the EPIC instrument with MOS1, MOS2 and pn, operating simultaneously. Therefore, the impact of the loss of CCD3 is zero for on-axis point sources and extended sources with radius smaller than 5.5 arcmin. For sources falling in CCD3 or for the extended emission of on-axis sources at distances larger than 5.5 arcmin, there is a 22% decrease in effective area (or 12% decrease in signal-to-noise ratio) over 14% of the field.
The combined impact of the two events from March 2005 and December 2012, which affected MOS1 CCD6 and CCD3, respectively, remains zero for on-axis point sources and a 12% reduction of the signal-to-noise in about 28% of the off-axis field of view for point-like or extended emission at radial distances larger than 5.5 arcmin.
• August 3, 2012: Astronomers have detected tell-tale luminosity fluctuations in the X-ray signal from a star that was torn apart and devoured by the supermassive black hole at the center of a distant galaxy. The fluctuations, which have a period of 200 seconds, originate from the innermost stable orbit around the black hole and represent the last signal sent by the debris of the disrupted star before disappearing beyond the black hole's event horizon. The discovery, based on data from ESA's XMM-Newton and the Japan/US Suzaku space observatories, has allowed astronomers to probe the details of matter accretion onto a supermassive black hole in the distant Universe for the first time. 167) 168)
- Black holes exist on a variety of scales, from the stellar-mass ones that derive from the collapse of massive stars to the supermassive black holes that reside at the center of most galaxies and have masses that are millions or even billions of times larger than the Sun's. Regardless of their mass, the phenomena that arise in the proximity of these extremely dense and compact objects due to their intense gravitational fields are quite similar. An effect of the accretion of the surrounding matter onto a black hole is the emission of radiation across the electromagnetic spectrum, which has been detected and studied extensively around stellar-mass and supermassive black holes alike. These observations allow astronomers to probe the behavior of gravity in its strongest regime and to test general relativity in a wide range of environments, both in our Galaxy (the Milky Way) and in more distant galaxies.
- A small fraction of the supermassive black holes hosted at the center of galaxies are undergoing 'active' accretion and feeding on a supply of gas at tremendous rates – these are the so-called AGN (Active Galactic Nuclei). However, the majority of supermassive black holes, including the one at the center of the Milky Way, are in a dormant state and only accrete matter on rare occasions, when a star happens to pass too close to it. In this case, matter on the side of the star facing the black hole experiences a stronger pull with respect to the other side, and this eventually tears the star apart. This phenomenon, referred to as tidal disruption, temporarily switches on the black hole's activity: debris from the shattered star starts orbiting around the black hole in a disc and part of it is rapidly accreted, causing a sudden boost in the luminosity of the galaxy's center, especially at the highest energies.

Legend to Figure 124: This illustration shows an artist's impression of Sw J1644+57, a supermassive black hole hosted at the center of a distant galaxy. The black hole, previously dormant, was temporarily switched on as a star passed too close to it. Torn apart by the black hole's gravitational pull, the star was tidally disrupted and its debris started orbiting around the black hole forming a disc. Part of the debris was rapidly accreted by the black hole, causing a sudden boost in the luminosity of the host galaxy's center, especially at the highest energies.
Originally detected as a GRB (Gamma-Ray Burst) by the NASA satellite Swift, the source, named Sw J1644+57, remained exceptionally bright for a few weeks after its discovery, unlike any other known GRB. After further observations, astronomers were able to link the flaring source to a star that was being disrupted and subsequently devoured by the supermassive black hole at the center of a distant galaxy.
Further observations performed with the ESA XMM-Newton and the Japan/US Suzaku X-ray observatories revealed that Sw J1644+57 exhibits what astronomers call quasi-periodic oscillations: luminosity fluctuations that occur in a regular fashion but are only seen for a certain period of time before disappearing. Quasi-periodic oscillations are known to arise in a very special site around a black hole: the so-called innermost stable circular orbit, which depends on the black hole's mass and spin and defines its range of action. At distances larger than this limiting orbit (indicated with a yellow circle in the illustration), matter can revolve around the black hole on stable trajectories, but anything located within this orbit will inexorably precipitate towards the black hole and be quickly accreted onto it.
• June 18, 2012: In June 2012, X-ray astronomy celebrates its 50th anniversary, following the discovery of the first extra-solar cosmic X-ray source in 1962. The XMM-Newton team recognizes the rich heritage from which XMM-Newton has grown and joins astronomers all over the world in the celebration. 169)
- XMM-Newton has observed the first cosmic X-ray source different than the Sun, Scorpio X-1, which was discovered in June 1962: Figure 125 shows the high-resolution the soft X-ray spectrum of Sco X-1, taken with the RGS instrument.

• December 20, 2011: Astronomers have discovered a very slowly rotating X-ray pulsar still embedded in the remnant of the supernova that created it. This unusual object was detected on the outskirts of the Small Magellanic Cloud, a satellite galaxy of the Milky Way, using data from a number of telescopes, including ESA's XMM-Newton. A puzzling mismatch between the fairly young age of the supernova remnant and the slow rotation of the pulsar, which would normally indicate a much older object, raises interesting questions about the origin and evolution of pulsars. 170) 171)
- The spectacular supernova explosion that marks the end of a massive star's life also has an intriguing aftermath. On the one hand, the explosion sweeps up the surrounding interstellar material creating a supernova remnant that is often characterized by a distinctive bubble-like shape, on the other hand, the explosion also leaves behind a compact object – a neutron star or a black hole. Since supernova remnants shine only for a few tens of thousands of years before dispersing into the interstellar medium, not many compact objects have been detected while still enclosed in their expanding shell.
- An international team of astronomers has now discovered one of these rarely observed pairs, consisting of a strongly magnetized, rotating neutron star – a pulsar – surrounded by the remains of the explosion that generated it.
- The newly found pulsar, named SXP 1062, is located at the outskirts of the Small Magellanic Cloud (SMC), one of the satellite galaxies of the Milky Way. SXP 1062 is an X-ray pulsar, part of a binary system in which the compact object is accreting mass from a companion star, resulting in the emission of copious amounts of X-rays. The astronomers first detected the pulsar's X-ray emission using data from ESA's XMM-Newton as well as NASA's Chandra space-based observatories. A later study of optical images of the source and its surroundings revealed the bubble-shaped signature of the supernova remnant around the binary system.
![Figure 126: This image is a composite view of the newly discovered X-ray pulsar SXP 1062 still embedded in the remnant of the supernova that created it. SXP 1062 accretes mass from its stellar companion, a massive, hot, blue 'Be' star, the two objects forming a Be/X-ray binary [image credit: ESA/XMM-Newton/L. Oskinova, University of Potsdam, Germany/M. Guerrero, Instituto de Astrofisica de Andalucia, Spain (X-ray); Cerro Tololo Inter-American Observatory/R. Gruendl & Y. H. Chu, University of Illinois at Urbana-Champaign, USA (optical)]](https://www.eoportal.org/ftp/satellite-missions/x/XMM-Newton_040722/XMM-Newton_Auto1A.jpeg)
• June 28, 2011: With a stroke of luck, astronomers using ESA's XMM-Newton X-ray observatory have observed a neutron star in a peculiar X-ray binary system undergoing an extremely rare, intense flare. This outburst of X-rays, which lasted about four hours, was due to a sudden increase in the rate at which the neutron star was accreting matter from its companion, a blue supergiant star. By monitoring this phenomenon in unprecedented detail, the data provide the first, substantive evidence to explain such luminosity variations in this type of binary system; the flare appears to be due to the ingestion of a massive clump of matter by the neutron star. 172) 173)
- Amongst the multitude of binary star systems that shine brightly in X-rays, those consisting of a neutron star and a blue supergiant star are especially puzzling, none more so than a rather enigmatic sub-class known as Supergiant Fast X-ray Transients (SFXTs). Although usually faint - typically about 10 000 times less luminous than the brightest X-ray sources in the sky - SFXTs occasionally undergo powerful flares that boost their luminosity, for a few hours, up to the levels of the brightest X-ray binaries known. New observations of one of these curious sources have now provided evidence to explain the origin of their very peculiar behavior.
- "To investigate these huge luminosity variations we need to observe SFXTs during a flare, but this has proven to be extremely hard due to the rarity and unpredictability of such events," explains Enrico Bozzo from the ISDC Data Center for Astrophysics at the University of Geneva, Switzerland. A team led by Bozzo planned to study one particular object, the binary system IGR J18410-0535, which had been observed to flare on more than one occasion over the past few years. However, given the extremely rare occurrence of such events, which happen at most a few times per year, the main goal of the observations was to probe the system in its normal quiescent state. "Imagine then our surprise when we realized that we had caught the source while it was flaring," he adds.
- Expecting it to be rather faint, as SFXTs are most of the time, the team had secured a long observation of the source with ESA's X-ray observatory, XMM-Newton, in order to probe its properties in detail. There was no indication whatsoever that an outburst would take place during their scheduled observations. "Our discovery was a truly lucky one, and not only because XMM-Newton happened to be looking at this object during the flare," notes Bozzo. In fact, the planned observation was also, by good fortune, of the right duration. The source was observed for 12.5 hours, enabling the astronomers to record the entire extent of the flare, which lasted about 4 hours, as well as to follow the source for a few hours after it returned to its normal, dormant state. As a result of this fortuitous circumstance, Bozzo and his colleagues were able to study the event in great detail and to infer the underlying cause for the rare and powerful flares.
- It is well known that, in the case of SFXTs, the neutron star does not accrete matter through a disc, because the wind flowing out of the companion is too diffuse and fast to form one. Instead, matter is transferred directly from the wind, 'raining' onto the neutron star from virtually all directions and then being funnelled by the magnetic field lines towards its poles. The matter accretion process releases X-rays, and any abrupt outburst of this radiation, such as the detected flare, must have something to do with changes in the accretion rate.

• March 9, 2011: Astronomers working with data from several observatories, including ESA's XMM-Newton, have discovered the most distant, mature galaxy cluster yet. The cluster is seen as it was when the Universe was only about a quarter of its current age. In contrast to other structures observed in the young Universe, this object is already in its prime, as is evident from its diffuse X-ray emission and evolved population of galaxies. This shows that fully-grown galaxy clusters were already in place this early in cosmic history. 174) 175)
- According to the most widely accepted theory, structure in the Universe grows in a hierarchical fashion: under the effect of gravity, smaller objects build up first, and then accrete into progressively larger ones. This is why galaxy clusters, the largest cosmic structures that are bound by gravity, are the last to form. When these enormous assemblies of galaxies, hot gas and dark matter appeared in the cosmos is still an open question, and the urge to resolve this question drives astronomers to observe galaxy clusters that are as distant as possible.
- Given that galaxy clusters are scarce, and even more so at high redshifts where they are still taking shape, these observations are challenging. "This is why discovering a galaxy cluster at a redshift of 2 feels like unearthing a rare and valuable gem," says Raphael Gobat from the Commissariat à l'Énergie Atomique (CEA), in France, who led the extensive study that revealed what appears to be the most distant galaxy cluster detected yet.
- CL J1449+0856, the newly-found galaxy cluster, is seen at an era when the Universe was only 3 billion years old — less than a quarter of its present age. "As well as being at a record-breaking distance, what makes this object rather unique is that it's not a proto-cluster undergoing formation, as are many that have been detected at high redshifts, but it is already mature, a proper galaxy cluster," adds Gobat.
- A signature of its advanced evolutionary state is the diffuse X-ray emission associated with the cluster, observed with ESA's XMM-Newton. "Only galaxy clusters that have had time to fully develop, collapsing under the influence of their own gravity, are visible in X-rays," explains Alexis Finoguenov from the Max-Planck-Institut für extraterrestrische Physik (MPE), in Germany, co-author of the paper in which the result is presented. The X-ray emission originates from the hot intra-cluster gas: subject to the cluster's gravitational potential, the gas is compressed and heated to temperatures of over 10 million Kelvin, and shines at X-ray wavelengths.
• In December 2010, the XXL survey, an XMM-Newton Very Large Program, has been granted time to map two extragalactic regions of 25 deg2, at a depth of ~5 x10-15 erg/cm2/s (using 10 ks observations). While the main goal of the project is to constrain the Dark Energy equation of state using clusters of galaxies 176) , it will also have lasting legacy value for cluster scaling laws and studies of AGNs and the diffuse XRB (X-Ray Baxkground). 177)
• October 14, 2010: Astronomers using XMM-Newton and other world-class X-ray telescopes have probed a curious source, which emits flares and bursts just like a magnetar but lacks the extremely high external magnetic field typical of these objects. The detection of this source, which could be powered by a strong, internal magnetic field hidden to observations, may mean that many 'ordinary' pulsars are dormant magnetars waiting to erupt. 178) 179)
- Massive stars remain objects of curiosity even well after their demise. Ending their lives in dramatic fashion, as supernova explosions, they leave a very dense and compact remnant behind - a neutron star or a black hole, depending on the mass of the star. These remnants, characterized by intense gravitational fields, are the source of some extremely energetic events and give rise to a variety of interesting phenomena which can be observed throughout the entire electromagnetic spectrum.
- Neutron stars, in particular, derive from the collapse of stars originally as massive as 8 to 25 times the mass of the Sun and they harbor magnetic fields a million times stronger than the strongest ones ever produced on Earth. Spinning neutron stars can be observed as pulsating sources -hence the name, pulsars- with exceptionally short periods, ranging from about one thousandth of a second to ten seconds. Powerful beams of electromagnetic radiation are created by jets of energetic particles that stream out above the magnetic poles of the star; the 'blinking' effect arises because the pulsar's magnetic dipole is not always aligned with its axis of rotation. Young pulsars rotate extremely fast but release rotational energy and slow down as they age: older pulsars have thus longer periods than younger ones. By measuring the rate at which a pulsar spins down it is possible to estimate the intensity of its surface dipolar magnetic field.
- Magnetars are a special class of pulsars that stand out from the crowd because of their striking characteristics: they have long rotations periods, occasionally undergo episodes of extremely enhanced emission (about 10–100 times the usual value) and produce intense, short bursts of X-rays and gamma-rays.

Legend to Figure 128: Magnetars are pulsars (spinning neutron stars) characterized by long rotations periods, occasional episodes of extremely enhanced emission (about 10–100 times the usual value) and intense, short bursts of X-rays and gamma-rays; these highly energetic events are presumed to be powered by an intense magnetic field.
• June 21, 2010: A long-sought-after emission line of oxygen, carrying the imprint of strong gravitational fields, has been discovered in the XMM-Newton spectrum of an exotic binary system composed of two stellar remnants, a neutron star and a white dwarf. Astronomers can use this line to probe extreme gravity effects in the region close to the surface of a neutron star. 180)
- Stellar remnants are the last evolutionary step of the life of stars which, after having burned their nuclear fuel, collapse into very compact and exotic objects - white dwarfs, neutron stars and black holes, depending on the mass of the stars. With an enormous mass contained in a very restricted space, these objects are extremely dense; in particular, neutron stars and black holes give rise to very strong gravitational fields and thus prove to be excellent testbeds for Einstein's theory of general relativity.
- Stars often come in pairs, and neutron stars and black holes are no exception, often being found as one component of a binary system. Due to the strong gravitational attraction that the compact remnant exerts on its companion, material from the latter flows onto the remnant forming an accretion disc. As the material in the disc spirals around the remnant, it is heated up to millions of degrees - because of internal friction - and produces copious amounts of X-rays. These systems are thus referred to as X-ray binaries.
- The object of this study, 4U 0614+091, is a very special X-ray binary, consisting of two remnants, namely a neutron star accreting mass from a white dwarf. The fact that the companion star is also a compact object is evident from the exceptionally short orbital period of the system: in fact, the two objects orbit around each other in about 50 minutes, which identifies the source as an UCXB (Ultra-Compact X-ray Binary ).

Legend to Figure 129: X-ray photons irradiating the disc are reflected by the material within it. As the gas in the disc contains a wealth of hot electrons, photons scattering off these electrons gain energy, resulting in a broadening of the spectral lines towards higher energies. Gravitational redshift and the relativistic Doppler effect broaden the spectral lines towards lower energies - a signature of the strong gravitational field of the neutron star.
• May 31, 2010: Surveying the sky, XMM-Newton has discovered two massive galaxy clusters, confirming a previous detection obtained through observations of the Sunyaev-Zel'dovich effect, the 'shadow' they cast on the Cosmic Microwave Background. The discovery, made possible thanks to a novel mosaic observing mode recently introduced on ESA's X-ray observatory, opens a new window to study the Universe's largest bound structures in a multi-wavelength approach. 181)
- Galaxy clusters are the largest gravitationally bound objects in the Universe. As such, they are extremely important probes of cosmic properties on very large scales, since they form in the densest knots of the large-scale structure, the cosmic web. Originally discovered as an excess density (or cluster) of galaxies located at the same redshift, hence the name, there is much more to these enormous structures than mere galaxies: in fact, only about one tenth of the entire mass of a galaxy cluster arises from galaxies (up to a thousand in the most massive cases), another tenth consists of hot gas, and the remainder can be attributed to dark matter.
- The gas that fills galaxy clusters is hot enough to emit X-rays — with a temperature of more than 10 million Kelvin, the gas is ionized and electrons scattering off ions are decelerated, emitting radiation in the process. From measurements of the X-ray luminosity of galaxy clusters and of the gas temperature, the total mass of these structures can be estimated. This yields clear evidence that clusters are indeed gravitationally bound structures and that their mass is dominated by the elusive and invisible dark matter.

Legend to Figure 130: X-ray emission (in pink) along with Sunyaev-Zel'dovich effect (SZE) contours (in white) of the massive galaxy cluster SPT-CL J2332-5358, overlaid on an optical g-, r- and i-band color composite image.
• April 28, 2010: One of the teams behind ESA's XMM-Newton X-ray mission has unveiled the latest edition of their 2XMM catalog. The newest incarnation boasts an additional 42 000 entries, ratcheting up the total to over a quarter of a million X-ray sources. This unprecedented cosmic X-ray library is a valuable resource allowing astronomers to explore the extreme Universe. 182)
- This latest edition of the 2XMM catalog, an unrivalled storehouse of information on X-ray sources, is the largest ever assembled. It is constructed through the serendipitous data acquired while piggy-backing on XMM-Newton's normal observing program, which is based on competitive bids from the astronomical community. XMM-Newton makes over 600 observations each year but the target object typically only takes up a tiny fraction of the field of view of 30 arcmin, equivalent to the diameter of the full Moon. Keen not to waste such an opportunity, the images are also searched for additional X-ray sources, with the findings being accumulated in the 2XMM catalogue for future reference. On average, an extra seventy sources additional to the main object of interest are found.
• January 20, 2010: Observations of faint and distant galaxy groups made with the European Space Agency's XMM-Newton observatory have been used to probe the evolution of dark matter. The results of the study are reported in the 20 January issue of The Astrophysical Journal. 184)
- Dark matter is a mysterious, invisible constituent of the Universe which only reveals itself through its gravitational influence. Understanding its nature is one of the key open questions in modern cosmology. In one of the approaches used to address this question astronomers use the relationship between mass and luminosity that has been found for clusters of galaxies which links their X-ray emissions, an indication of the mass of the ordinary (baryonic) matter alone, and their total masses (baryonic plus dark matter) as determined by gravitational lensing.
- To date the relationship could only be established for nearby clusters. New work by an international collaboration, including the Max Planck Institute for Extraterrestrial Physics (MPE), the Laboratory of Astrophysics of Marseilles (LAM), and Lawrence Berkeley National Laboratory (Berkeley Lab), has made major progress in extending the relationship to more distant and smaller structures than was previously possible.
- To establish the link between X-ray emission and underlying dark matter, the team used one of the largest samples of X-ray-selected groups and clusters of galaxies, produced by the ESA's X-ray observatory, XMM-Newton.

Legend to Figure 132: The Cosmic Evolution Survey (COSMOS) is an astronomical survey designed to probe the formation and evolution of galaxies as a function of cosmic time (redshift) and large scale structure environment. The survey covers a 2 square degree equatorial field with imaging by most of the major space-based telescopes, including Hubble and XMM-Newton, and a number of ground-based telescopes.
• On December 10, 2009 the XMM-Newton spacecraft was 10 year on orbit. Over the course of the past 10 years XMM-Newton, in tandem with NASA's Chandra X-ray Observatory, has revolutionized the field of X-astronomy. The complementary capabilities of the two X-ray observatories, with superb spectroscopy from XMM-Newton and exquisite imaging from Chandra, have provided X-ray astronomers with the scientific probes required to investigate the physical conditions and mechanisms underpinning the most energetic and violent processes in the Universe. 185) 186)
- As the first decade of XMM-Newton draws to a close, scientists are already preparing for the future. While XMM-Newton has answered many of the questions that it was designed to address, the extraordinary view of the X-ray Universe that it has provided has also raised new questions, such as, how do black holes and matter behave under extreme conditions, can the nature of dark matter and dark energy be constrained by studying the evolution of galaxy formation, and how are chemical elements created and dispersed. The fact that the XMM-Newton spacecraft and instruments are in excellent condition and able to continue to operate for another decade means that this astronomical workhorse is well placed to pursue new challenges.
• July 1, 2009: Astronomers using ESA’s XMM-Newton X-ray observatory have discovered a black hole weighing more than 500 solar masses, a missing link between lighter stellar-mass and heavier supermassive black holes, in a distant galaxy. This discovery is the best detection to date of a new class that has long been searched for: intermediate mass black holes. 187)
- Stellar-mass black holes (about three to twenty times as massive as the Sun) and supermassive black holes (several million to several thousand million times as massive as the Sun) have long been known to exist. Because of the large gap between these two extremes, scientists have speculated the existence of a third, intermediate class of black holes, with masses between a hundred and several hundred thousand solar masses.
- Up until now, scientists were unable to confirm that this elusive intermediate class actually existed.
- Farrell’s team at the University of Leicester were analyzing archived data obtained by XMM-Newton, looking for neutron stars and white dwarves, when they stumbled upon a most peculiar object that was observed on 23 November 2004.
- Called HLX-1 (Hyper-Luminous X-ray source 1), it lies towards the outskirts of the galaxy ESO 243-49, approximately 290 million light-years from Earth. If it is indeed located in this distant galaxy, HLX-1 is very luminous in X-rays; peaking at 260 million times the luminosity of the Sun.

Legend to Figure 133: Illustration of HLX-1 (blue star to the upper left hand side of the galactic bulge). HLX-1, located on the outskirts of the spiral galaxy ESO 243-49, is the strongest candidate to- date of intermediate-mass black holes.
• Nov. 14, 2008: X-ray and gamma-ray data from ESA’s XMM-Newton and Integral orbiting observatories has been used to test, for the first time, the physical processes that make magnetars, an atypical class of neutron stars, shine in X-rays. 188)
- Neutron stars are remnants of massive stars (10-50 times as massive as our Sun) that have collapsed onto themselves under their own mass. Made almost entirely of neutrons (subatomic particles with no electric charge), these stellar corpses concentrate more than the mass of our Sun within a sphere about 20 km in diameter.
- They are so compact that a teaspoon of neutron star stuff would weigh about one hundred million tons. Two other physical properties characterize a neutron star: their fast rotation and strong magnetic field. - Magnetars form a class of neutron stars with ultra-strong magnetic fields. With magnetic fields a thousand times stronger than that of ordinary neutron stars, they are the strongest known magnets in the cosmos.

Legend to Figure 134: X-ray and gamma-ray data from ESA’s XMM-Newton and Integral orbiting observatories has been used to test, for the first time, the physical processes thought to lie behind the emission of magnetars, an atypical class of neutron stars with ultra-strong magnetic fields.
• October 23, 2008: XMM-Newton, ESA’s X-ray observatory, has re-established communication contact with Earth, showing that the spacecraft is safe and fully under control. The news was confirmed this morning by the mission control team at ESA’s European Space Operations Center (ESOC) in Darmstadt, Germany. 189)
- Radio contact was re-established on Wednesday 22 October around 18:00 CEST (Central European Summer Time). This followed an unexpected radio silence from the spacecraft which started on 18 October 2008, when XMM-Newton, coming out of a nominal period of non-radio visibility along its orbit around Earth, did not succeed in sending the expected signal to Earth.
- Fearing the worst, teams at ESOC and other ESA establishments, supported by industry, immediately started studying the situation and performing the first attempts of recovery. The first signs of hope arrived on Monday evening when images obtained by the Starkenburg Observatory in Germany confirmed that the spacecraft was intact and did not give any sign that it was tumbling. This fact was also confirmed by many other ground observatories from all over the world that had answered XMM-Newton’s call for help.
- The initial hopes were then confirmed, when ESOC ground controllers, using ESA’s 35 m ground station in New Norcia (Western Australia), managed to establish a feeble two-way communication path to the spacecraft. This suggested a failure in the on-board Radio Frequency (RF) switch – an important clue for the experts that could then focus on more targeted solutions.
- Once these solutions were simulated and validated on ground, the final recovery action started. Ground controllers made use of the NASA 34 m DSN ( Deep Space Network) ground station in Goldstone (California), which is located in a favorable position for times when XMM-Newton is closest to Earth (perigee passage). They sent a command that allowed the RF switch to go back to its last working position and then managed to get radio contact with the spacecraft with ESA’s 15 m ground station at ESA/ESAC (European Space and Astronomy Center) near Madrid, Spain.
- “XMM-Newton is now safe and fully under control,” said Dietmar Heger, XMM-Newton Deputy Spacecraft Operations Manager at ESOC. “It’s been a thrilling moment for our team. We even feared the spacecraft could be lost, but hard team work and a good star helped us turn this into a new success story for ESA.”
- “XMM-Newton has been in orbit for almost nine years, investigating the violent Universe as never before and providing discovery after discovery” added Arvind Parmar, XMM-Newton Mission Manager at ESA/ESTEC (European Space Research and Technology Center) and Head of the Astronomy Science Operations Division at ESAC. “It’s a big relief to know we can still count on this great spacecraft. We thank all the teams which have worked non-stop in the past days to make this possible, and our colleagues at NASA who made their 34 m station available.”
• August 25, 2008: ESA’s orbiting X-ray observatory XMM-Newton has discovered the most massive cluster of galaxies seen in the distant Universe until now. The galaxy cluster is so big that there can only be a handful of them at that distance, making this a rare catch indeed. The discovery confirms the existence of dark energy. 190)
- The newly-discovered monster, known only by the catalogue number 2XMM J083026+524133, is estimated to contain as much mass as a thousand large galaxies. Much of it is in the form of 100-million-degree hot gas. It was first observed by chance as XMM-Newton was studying another celestial object and 2XMM J083026+524133 was placed in a catalog for a future follow-up.
- Georg Lamer, AIP (Astrophysikalisches Institut Potsdam), Germany, and a team of astronomers discovered the record-breaking cluster as they were performing a systematic analysis of the catalogue. Based on 3500 observations performed with XMM-Newton's European Photon Imaging Camera (EPIC) covering about 1% of the entire sky, the catalogue contains more than 190 000 individual X-ray sources. The team were looking for extended patches of X-rays that could either be nearby galaxies or distant clusters of galaxies.

The optical image that confirmed that 2XMM J083026+524133 is a distant cluster of galaxies, taken by the Large Binocular Telescope in Arizona. The X-ray emission from the cluster of galaxies is shown in blue at the center of the image. The individual galaxies in the cluster are the small dots inside the blue glow.
• June 23, 2008: XMM-Newton has, for the first time, detected signals from both stars of a binary pulsar system in X-rays, unveiling a scientific goldmine. Each star of the closely-packed system is a dense neutron star, spinning extremely fast, radiating X-rays in pulses. - The binary pulsar PSR J0737-3039 was first spotted by astronomers in 2003 in radio wavelengths. X-rays can be used to probe deeper and study the system more thoroughly. 191) 192)
- To see two pulsars orbiting each other in a binary system is extremely rare in itself. PSR J0737-3039 contains a slowly-rotating ‘lazy’ neutron star (pulsar B) orbiting a faster and more energetic companion (pulsar A).
- Each pulsar or neutron star is the fast-rotating, dead heart of a once-massive star. “These stars are so dense that one cup of neutron star-stuff would outweigh Mt. Everest,” says Alberto Pellizzoni, lead author of the article where the results are reported. “Add to that the fact that the two stars are orbiting really close to each other, separated by only 3 light-seconds, about three times the distance between Earth and the Moon.”
- The fundamental physical processes involved in these extreme interactions are a matter of debate among theoretical physicists. But now, with XMM-Newton's observations, scientists have gained new insight, providing a new experimental setting for them. In X-rays, it will be possible to study the subsurface and magnetospheres of the stars as well as the interaction between the two in that close, heated environment.
• December 21, 2007: XMM-Newton has detected periodic X-ray emission, or the pulsed heartbeat of a weird new type of star. Collecting the X-rays from the so-called rotating radio transient has confirmed the nature of the underlying celestial object and given astronomers a new insight into these exotic objects. 193)
- The observations were made using XMM-Newton’s EPIC (European Photon Imaging Camera), which targeted the celestial object RRAT J1819–1458. Astronomers observed the object for around 12 hours and detected pulsations in the X-ray data that show the source to be rotating once every 4.26 seconds.
- Previously, astronomers had only seen radio outbursts from this object. It erupts every three minutes or so with a brief burst of radio emission lasting just 3 ms (milliseconds). Such behavior defines the object as a RRAT (Rotating Radio Transient).

• Nov. 14, 2007: In recognition of their superb scientific output, the mission operations of European flagship x-ray and gamma-ray observatories, XMM-Newton and Integral, have been extended until 31 December 2012. The extension of both missions, which have been producing an incessant flow of science results since launch, was announced this week after a unanimous vote at a meeting of ESA’s Science Program Committee. 194)
- In the last two years XMM-Newton has made breakthrough observations of a wide variety of compact objects, such as the first detection of an intermediate-mass black hole in globular cluster NGC 4472. This has direct implications for the formation and evolution theories of globular clusters in general.
- Thanks to its sensitivity at high energies, XMM-Newton has made the first and only direct probing of the central regions near a black hole, by sampling the presence of iron and the variability of its spectral fingerprints. The satellite’s observations have also been fundamental in helping understand the physics of heavy sub-atomic matter (‘baryonic’) in clusters of galaxies and on studying the dark matter component in clusters.
- XMM-Newton has given the first strong indication that very faint AGN (Active Galactic Nuclei) are similar to the 'normal' AGN population, and measured for the first time the size of the emission region of an AGN.
- Other major results include the progress in understanding the link between X-ray emission and luminosity of stars, as well as the relation between the X-ray emission and processes such as star accretion or collisions.
- The satellite has also discovered the remnants of a new class of supernovae within the so-called ‘Ia type’, which are used as standard reference, or ‘candles’, to determine stellar luminosity.
- XMM-Newton has also revealed X-ray emission in the Martian exosphere - the first definite detection of X-ray emission induced by exchange of electrical charges from the exosphere of another planet.
• Sept. 7, 2007: ESA released the largest catalog of X-ray sources ever, referred to as 2XMM, complied from 6 years of observations of the XMM-Newton mission. The catalog contains 247,000 X-ray source detections which relate to 192,000 unique X-ray sources, making it the largest collection of objects ever observed in the X-ray spectrum. 195)

Legend to Figure 137: Covering 360 square degrees of the sky, 2XMM complements deeper Chandra and XMM-Newton small area studies and covers astronomical objects that dominate the X-ray background spread across the Universe. It provides a unique dataset for generating large, well-defined samples of various types of high-energy astrophysical objects. X-ray selection is the most efficient tool to pinpoint such objects.
XMM-Newton has, for the first time, detected signals from both stars of a binary pulsar system in X-rays, unveiling a scientific goldmine. Each star of the closely-packed system is a dense neutron star, spinning extremely fast, radiating X-rays in pulses. The binary pulsar PSR J0737-3039 was first spotted by astronomers in 2003 in radio wavelengths. XMM-Newton discovered X-ray emission from both pulsars in October 2006. 197)
• Nov. 27, 2001: Probably the most detailed analysis of the composition and dynamics of the supernova remnant Cassiopeia-A has been presented at the symposium 'New Visions of the X-ray Universe in the XMM-Newton and Chandra era' which is taking place this week at the ESA/ESTEC, Noordwijk in the Netherlands.
Cassiopeia A (Cas-A) is a young shell-shaped supernova remnant some 15 light years in diameter situated some 10,000 light years away. It is the remains of a massive star which, having exhausted all its hydrogen fuel, exploded 320 years ago. The core of such a collapsing star can give rise to a neutron star or black hole. Its external parts are blown apart projecting stellar material, glowing in X-rays, into the surrounding interstellar medium. The stellar material contains many heavy elements which have been forged from lighter elements in the progenitor star, and during the explosion process. All the chemical elements in our human bodies have their origins in such stellar explosions and the resulting primordial broth (Figure 138). 198)

• Feb. 9, 2000: The first pictures from ESA's new X-ray space observatory fully demonstrate the capabilities of the spacecraft's telescopes and its science instruments. The images were officially presented on 9 February at the XMM-Newton Science Operations Center in Villafranca, Spain. — The images were obtained between 19-25 January at the very start of the science payload commissioning process. The spacecraft viewed three regions of the sky: part of theLMC ( Large Magellanic Cloud ), the Hickson Cluster Group 16 (HCG-16), and the star HR 1099. These targets were chosen because they all present a variety of X-ray extended and point sources and are very interesting regions. 200)
The Large Magellanic Cloud is about 20 ,000 light years in diameter. Situated 160,000 light years from Earth, it is one of two irregularly shaped galaxies that are easily seen with the naked eye in the southern hemisphere. These galaxies are satellites of the Milky Way and appear to be slowly spiralling into our own Galaxy.
- The X-ray color image (Figure 139) made with the EPIC camera on XMM shows part of a small companion galaxy to the Milky Way, the Large Magellanic Cloud, where stellar explosions are releasing newly manufactured elements, and new stars are being formed. The image is made so as to reveal the temperature of the X-ray emitting medium, with blue indicating the hottest regions; green the intermediate temperatures and red the coldest regions. Most of the 'blue' X-rays have not been observed before, and it is the collecting power of XMM that enables these observations. 201)

- XMM brings to bear the largest X-ray telescopes in space, and this is shown here. These colliding galaxies are 170 million light years away, and are clearly visible to EPIC. In the background more than a hundred faint X-ray sources are seen, most of these are new detections by XMM. 202)

- X-ray image of the field around the bright star HR1099 (Figure 141). This X-ray image also illustrates the collecting power of XMM, as many of the other X-ray sources in the image were hitherto unknown, or allow more detailed studies of these objects than before. HR1099 is a nearby star with a hot corona which is very active in X-rays. 203)

Sensor Complement
EPIC (European Photon Imaging Camera)
Three cameras, one at the primary focus of each mirror module, were produced by a consortium of ten institutes in four nations: the UK, Italy, France and Germany. The EPIC Principal Investigator (PI) is Dr. Steven Sembay of the University of Leicester, UK. He took over from Prof. Martin Turner of the X-ray Astronomy Group at Leicester University, who was the EPIC PI up to 6 May 2009. One of the cameras uses a new type of CCD (pn) developed by the Max Planck Institute of Extraterrestrial Physics in Garching and the Astronomical Institute in Tübingen, both in Germany.
EPIC comprises a set of three X-ray CCD cameras. Two of the cameras contain MOS CCD arrays (referred to as the MOS cameras). They are installed behind the X-ray telescopes that are equipped with the gratings of the RGS. The gratings divert about half of the telescope incident flux towards the RGS detectors such that about 44% of the original incoming flux reaches the MOS cameras. The EPIC instrument at the focus of the third X-ray telescope with an unobstructed beam; uses pn CCDs and is referred to as the pn camera (Figure 144).
The EPIC cameras perform extremely sensitive imaging observations over the telescopes FOV (Field of View) of 30 arcmin and in the energy range from 0.15 to 15 keV with moderate spectral (E/ΔE ~ 20-50). All EPIC CCDs operate in photon counting mode with a fixed, mode dependent frame read-out frequency. 205) 206)


EPIC MOS CCDs: The MOS CCDs, EEV type 22, have 600 x 600 pixels, each 40 µm x 40 µm; they are frame-transfer devices and front illuminated. One pixel covers 1.1 arcsec. The third phase electrode is open so that 40% of the area is only covered by 400 Å of silicon oxide. This improves the low energy response, giving appreciable sensitivity down to 150 eV. There being no buried channel makes this part of the pixel immune to channel damage such as that caused by soft protons interacting just below the surface of the pixel. This, combined with the rather thicker oxide and poly-silicon that covers the buried channel, contributes to the relative radiation hardness of the devices to soft protons.

The full frame integration time is 2.6 seconds, governed by the time taken to read out the 600 x 600 pixels with sufficiently low noise (<5 electrons). The CCDs are read out in sequence. The central CCD can be commanded independently of the others, which are commanded in pairs; the modes can be applied to the central CCD as described in Table 5 and Figure 145.
Central CCD mode | Imaging area pixels (arcmin) | Time resolution (s) |
Full | 600 x 600 (11 x 11) | 2.6 |
Large window | 300 x 300 (5.5 x 5.5) | 0.6 |
Small window | 100 x 100 (1.8*1.8) | 0.3 |
Timing | None (1.8 arcmin projection) | ~10-3 |
The CCDs are cooled passively and maintained at their operating temperature using a three-stage radiator system combined with heaters. The minimum design temperature for the cooling system is –130 °C. There is provision for de-icing the radiators and CCDs by raising the temperature to near 0ºC and for annealing the CCDs by raising their temperature to +120ºC. Neither of these operations has so far needed to be performed. The nominal operating temperature of the CCDs at launch was –100 ºC, and this is maintained to within ± 0.5 º by the control electronics.
EPIC PN CCDs: The principle of sideward depletion in high resistivity silicon is the basis of a large variety of novel silicon detectors, such as silicon drift detectors, controlled drift detectors, active pixel sensors — and pn-CCDs. The essential part of the EPIC PN detector is the 10 cm silicon wafer divided in an array of 12 monolithically implanted pn-CCDs, developed and manufactured in a dedicated semiconductor laboratory . The array has a total effective size of 6 cm x 6 cm. The CCDs are arranged in four quadrants of three CCDs each. For redundancy reasons, each quadrant can be considered as a separate unit: it has its own power supplies, back contact, preamplifiers and event analyzer electronics and can be operated independently from the others. The single CCD has a dimension of 3 cm x 0.98 cm with pixels of 150 µm x 150 µm arranged in 200 rows and 64 channels. The pixel size corresponds to an angular resolution element of 4.1 arcsec. The 64 read out anodes of each CCD are connected via on chip JFETs (Junction Field Effect Transistor) to a 64 channel charge sensitive amplifier (CAMEX64B). The 12 x 64 output channels of the CCDs as well as the supply and bias voltages are connected via bond wires to a multilayer printed circuit board, which carries the charge sensitive amplifiers as well as filter circuits for supply and bias voltages. 207)


CCD mode | Imaging area pixels (arcmin) | Time resolution (s) |
Full | 384 x 376 (26 x 27) | 73 |
Large window | 384 x 198 (26 x 13.6) | 48 |
Small window | 64 x 64 (4.4 x 4.4) | 5.7 |
Timing | None (4.4 arcmin projection) | 0.03 |
Several read out modes can be selected for the pn-camera (Figure 147) to adjust the performance to the observation requirements (source flux). The full frame and window modes are the imaging modes. Timing and burst mode achieve better time resolutions at the expense of the coordinate in shift direction. In full frame mode, all 12 CCDs are read out sequentially within 70.25 ms, which corresponds to the time resolution in this mode. A CCD remains sensitive also during its read out cycle. This causes the so called 'out-of-time' events. These are events arriving during read out phase, when the charge content of the CCD is shifted to the read out anodes. The out-of-time events are distributed along the shift direction and get therefore a wrong coordinate in this direction. In full frame mode their fraction of 6.6% is given by the ratio between the read out time of one CCD and the read out time of all CCDs including 'warm up' times for preamplifiers.
EPIC Filter Wheel: Because CCDs are light sensitive, two kinds of light filter are provided. Their design is a compromise between the need to prevent optical and UV photons from reaching the CCD plane, and the need to absorb as few X-ray photons as possible, especially at the lowest X-ray energy. The thin and medium filters comprise an unsupported polyimide film, 160 nm thick, on with a single layer of aluminum; 40 nm on the thin filter and 800 nm on the medium. The transmission of visible light is approximately 10-2 and 10-4, respectively. The thick filter is constructed on an unsupported polypropylene film 330 nm thick, with 160 nm of aluminum and 40 nm of tin to block the UV that would otherwise be transmitted by the polypropylene. Because of the large diameter (~65 mm) and delicacy of these filters, it was felt unwise to launch them other than under vacuum, thus minimizing the acoustic load, transferred to the filter from the rocket. Late in the campaign, a leak developed in one of the cameras that prevented the vacuum from being maintained in the long period between last access for pumping and the launch. This camera was filled with helium at atmospheric pressure with a blow-off valve to vent the camera during ascent. This system worked effectively to reduce acoustic load (cf. possible load with air) and the filters suffered no damage during launch. During radiation belt passage, and at all other times deemed unsafe for operation, the filter wheels are kept in the closed position.. There are ‘Closed-Cal’ observations used to monitor the long-term degradation of the CCD performance, by closing the filter wheel with a calibration source illuminating the CCDs. Once the radiation level is deemed to be safe, the filter wheel is moved to the appropriate filter position and the observation begins.
RGS (Reflection Grating Spectrometer)
Two of XMM-Newton's three mirror modules are equipped with an RGS grating array that disperses about 40 per cent of the light to an RGS detector in a secondary focus. The RGS Principal Investigator is Dr. Jelle Kaastra of the High-Energy Astronomy division SRON (Space Research Organization Netherlands), Utrecht, The Netherlands. He took over from Prof. Bert Brinkman, SRON, Utrecht, The Netherlands, who was the first RGS PI until 1 April 2002.
Optical design: The RGS design is illustrated in Figure 148. It incorporates an array of reflection gratings (RGA, aka grating stack) placed in the converging beam of an XMM-Newton telescope mirror module, this is done for two out of the three mirror modules on XMM-Newton. The grating stack consists of 182 precisely aligned reflection gratings which in total intercept about half the light emanating from the telescope. The undeflected light passes through and is collected by the EPIC instrument in the telescope focal plane. The individual gratings are located on a toroidal Rowland surface, formed by rotating the Rowland circle about an axis passing through the telescope and spectroscopic foci, as illustrated in the left panel of Figure 148. The gratings are slightly trapezoidal, since their edges lie along rays converging on the telescope focus.
While the field of view in the cross dispersion direction is determined by the width of the CCDs, the spatial resolution in this direction is largely determined by the imaging properties of the mirror. The situation in the dispersion direction is more complex as the source extent affects the wavelength resolution.
Nine large format back-illuminated CCDs are located on the Rowland circle to detect the dispersed spectra in single photon counting mode. The position of the X-ray on the detector is related to the X-ray wavelength through the dispersion equation. First, second and higher order spectra are overlapping on the detectors, but are easily separated by using the inherent energy resolution of the CCDs.
RGA (Reflection Grating Array): A RGA contains 182 identical gratings. The gratings are mounted at grazing incidence in the in-plane, or classical configuration, in which the incident and diffracted X-rays lie in a plane that is perpendicular to the grating grooves. Because the beam is converging, the gratings are oriented so that the angle of the incident X-ray at the center of the grating, α, is the same for all gratings in the array. In addition, the gratings all lie on the Rowland circle, which also contains the telescope focus and the spectroscopic focus for the blaze wavelength. In this configuration, aberrations, which would otherwise be introduced by the arraying, are eliminated. The telescope aperture is filled by rotating the Rowland circle about an axis passing through the telescope and spectroscopic blaze foci. In all, each RGA contains six rows of gratings.

Each grating measures about 10 cm x 20 cm (Figure 149). These large gratings need to be very flat in the long (i.e. dispersion) direction, since any non-flatness translates directly into a degradation of the resolution. At the same time, the grating substrates need to be very thin in order to minimize the obstruction of the direct beam by the gratings. The grating substrates consist of 1mm SiC face sheets with five stiffening ribs at the back, running in the direction of the X-ray beam (Figure 149). The face sheets are fabricated to 1 λ (634.8 nm) and 10 λ flatness in the long and the short direction, respectively. The gratings are replicated from a mechanically ruled master and are covered with a 200 nm gold coating. The groove density varies slightly (± 10 %) over the length of the gratings, to correct for aberrations associated with the converging beam. The groove density is ~646 grooves/mm at the center.

The grating array support structure is made out of vacuum hot-pressed beryllium, which was selected for its low specific mass and good stability over the operational temperature range (10-30 ºC). To obtain the desired resolution, it is essential that all gratings are properly aligned (with 1 µm tolerance on the position of any grating corner).
RGS Focal plane camera: The dispersed spectrum is integrated on nine large format back illuminated CCDs. These nine CCDs are mounted in a row, following the curvature of the Rowland circle (see top two panels of Figure 150). To reduce the dark current and improve general performance the CCD’s are cooled to -80 ºC. An increase in CCD charge transfer inefficiency (CTI) due to radiation damage can be reduced by lowering the temperature to -120 ºC. Cooling is accomplished by a two-stage radiator, facing deep space. The CCD bench is housed internally to three nested thermal shells around the CCD bench where the first shield also contains four internal calibration sources. The nine CCD chips are back-illuminated EEV devices with an image and storage section of 384 x 1024 pixels each and a pixel size of 27 µm by 27 µm and the length of the CCD assembly (253 mm) covers the first order dispersion of the 0.6 – 3.8 nm wavelength range. The instrument can be operated in a spectroscopy, high time resolution and diagnostic mode.

OM (Optical Monitor)
OM is an optical/ultraviolet telescope, co-aligned with the main X-ray telescope, gives the XMM-Newton mission a multi-wavelength capacity. The Mullard Space Science Laboratory (MSSL) UK has supplied this 30 cm aperture Ritchey-Chrétien telescope (with a 180-600 nm spectral range). The OM Principal Investigator is Prof. Keith Mason.
The Optical/UV Monitor Telescope (XMM-OM) is mounted on the mirror support platform alongside the X-ray mirror modules. It provides coverage between 170 nm and 650 nm of the central 17 arcmin square region of the X-ray field of view, permitting routine multi-wavelength observations of XMM targets simultaneously in the X-ray and ultraviolet/optical bands A schematic of the XMM-OM is shown in Figure 151. It consists of two modules which are physically separated on the spacecraft; the telescope module and dual redundant digital electronics modules. The Telescope Module contains the telescope optics and detectors, the detector processing electronics and power supply. The Digital Electronics Module houses the ICU (Instrument Control Unit), which handles communications with the spacecraft and commanding of the instrument, and the Data Processing Unit, which preprocesses the data from the instrument before it is telemetered to the ground.

The telescope is of a Ritchey-Chrétien design and has a clear aperture of 30 cm. The f/ratio of the primary mirror is f/2.0, which is modified by the secondary to f/12.7, i.e. a focal length of about 3.8 m. The light beam is intercepted by a 45º flat mirror, located behind the primary mirror, which can be rotated to direct the beam to one of two redundant filter-wheel/detector assemblies. The format of the detector is 2048 x 2048 pixels with each pixel 9.5 µm square. The field of view is 24 arcmin on the diagonal. In order to flatten the intrinsically curved focal plane, the detector window has been made concave (thinner at the center), and the filters are weakly figured.
The detector is a microchannel plate (MCP)-intensified CCD (MIC). Incoming photons are converted into photoelectrons in an S20 photocathode deposited on the inside of the detector window. The photoelectrons are proximity focused onto a stack of three microchannel plates, which amplifies the signal by a factor of a million, through a bias of ~1.8 kV. The resulting electrons are converted back into photons by a P46 phosphor screen. Light from the phosphor screen is passed through a fiber taper which reducing the image scale to compensate for the difference in physical size between the microchannel plate stack and the fast-scan CCD used to detect the photons.
In each detector there is a filter wheel. The filter wheel has 11 apertures, one of which is blanked off to serve as a shutter, preventing light from reaching the detector. Another seven filter locations house lenticular filters, six of which constitute a set of broad band filters for color discrimination in the UV (UVW1, UVM2, UVW2) and optical (U, B, V) between 180 nm and 580 nm. The seventh is a "white light" filter which transmits light over the full range of the detector to give maximum sensitivity to point sources (Figure 152). The remaining filter positions contain two grisms, one optimized for the UV and the other for the optical range, and a x4 field expander.

The signal from the CCD detector is processed by fast electronics near the detector head to extract information which is then transmitted to the DPU (Data Processing Unit) in the DEM (Digital Electronics Module). Each DEM contains an ICU (Instrumental Control Unit) and a DPU. The ICU commands the XMM-OM and handless communications between the XMM-OM and the spacecraft. The DPU is an image processing computer that digests the raw data from the instrument and applies a non-destructive compression algorithm (“tiered block word length” type) before the data are telemetered to the ground via the ICU. The DPU supports two main science data collection modes, which can be used simultaneously. The DPU autonomously selects up to 10 guide stars from the full OM image and monitors their position in detector coordinates at intervals that are typically set in the range 10-20 seconds, referred to as a tracking frame. These data provide a record of the drift of the spacecraft during the observation accurate to ~ 0.1 arcsec.
The OM telescope module consists of a stray light baffle and a primary and secondary mirror assembly. The separation of the primary and secondary mirrors is critical to achieving the image quality of the telescope. The separation is maintained to a level of 2 µm by Invar support rods that connect the secondary spider to the primary mirror mount, and by maintaining an isothermal condition through distributing the detector electronics heat with heat pipes.
Ground Segment
The ground segment consists of all of the infrastructure and systems needed on Earth to communicate with, monitor and control the XMM satellite in real time, as well as to gather, process and archive the scientific data harvested by the X-ray cameras on board.

The major components of the XMM ground segment are:
1) The ground stations to track the satellite and communicate at S-band with the on-board transponders. During the operational scientific part of the mission, two ESA ground stations are used: Perth in Australia and Kourou (the ‘Diane’ station) in French Guiana. These stations were selected as offering near-complete coverage of the XMM orbit, with its apogee in the Southern-Hemisphere. During XMM’s first 10 days in space – the so-called LEOP (Launch and Early Orbit Phase) – a third ESA station will be used in addition, namely Villafranca in Spain.
2) The MOC (Mission Operations Center) located at ESOC in Darmstadt (Germany). The MOC is responsible for monitoring and controlling the satellite: all telecommands will be sent from the MOC, and all telemetry will be received at the MOC.
The main elements of the Mission Operations Center are:
• The XMCS (XMM Mission Control System), in charge of receiving, decoding and processing the telemetry, as well as assembling the telecommands to the satellite.
• The XMM Simulator (called the MOC-SIM), a software model of the satellite platform, used for pre-validation of commands and procedures.
• The FDS (Flight Dynamics System), in charge of orbit determination and attitude reconstitution. The FDS also defines all of the attitude and orbit control maneuvers in an optimal way to maximize the duration of the scientific observations, whilst still respecting the XMM-imposed in-orbit constraints, such as avoidance of bright celestial bodies in the telescope field of view.
• The SOC (Science Operations Center) at ESAC ((European Space Astronomy Center), is located at Villafranca del Castillo near Madrid, Spain. The SOC is responsible for preparing all scientific observations, and analyzing and processing the corresponding scientific data. The main elements of the SOC are:
- The XMM Science Control System (XSCS) in charge of defining the planning of the scientific observations and monitoring their execution
- The XMM Simulator (called the SOC-SIM), a software model of the satellite experiments, used for validation of ground procedures, as well as validation of instrument on-board software
• The XMM Archive Management System (AMS), which stores and allows retrieval of all XMM data and associated products.
• The XMM SSC ( Science Survey Center) at Leicester University, United Kingdom, also plays a role. It performs pipeline processing of all XMM science data to identify and categorize all X-ray sources detected by XMM instruments.
- The XMM-Newton SSC Survey Science Center consortium was selected by ESA in early 1996. The SSC is an international collaboration involving a consortium of 8 institutions in the UK, France and Germany, together with 7 Associate Scientists. The SSC's role in facilitating the exploitation of the XMM-Newton serendipitous survey. 208)
Normal operations allow coverage of the observational periods of spacecraft orbit with only two ground stations (Perth and Kourou), however there is a seasonally varying gap close to spacecraft apogee of order 1 hour, during which no science operations can be conducted. This implies that uninterrupted exposures of about 65 ks can be implemented at the moment. It is anticipated that by the end of the year 2000, full orbit coverage can be achieved by using an additional operational antenna (Ref. 2).
Packets of science data from the 6 instruments, are multiplexed with their housekeeping data and spacecraft telemetry, and transmitted to ground at a rate of 64 kbit/s maximum. Spacecraft data are merged with earth reception time references, and transmitted via ground telecommunications links to the MOC (Mission Operations Center) at Darmstadt. The MOC monitors real-time payload health, performs commanding of operations, and provides additional analysis of spacecraft data to provide (for example) the Attitude History Files for each science observation. The science data are further transmitted to the SOC (Science Operations Center) at Villafranca, where science Quick Look Analysis is performed. The SOC then processes the data into the ODFs (Observation Data Files) for transmission to the science community.
The XMM-Newton project is using the ground stations at Perth (Australia), Kourou (French Guiana), Villafranca (Spain) and Santiago (Chile), according to Ref. 153).
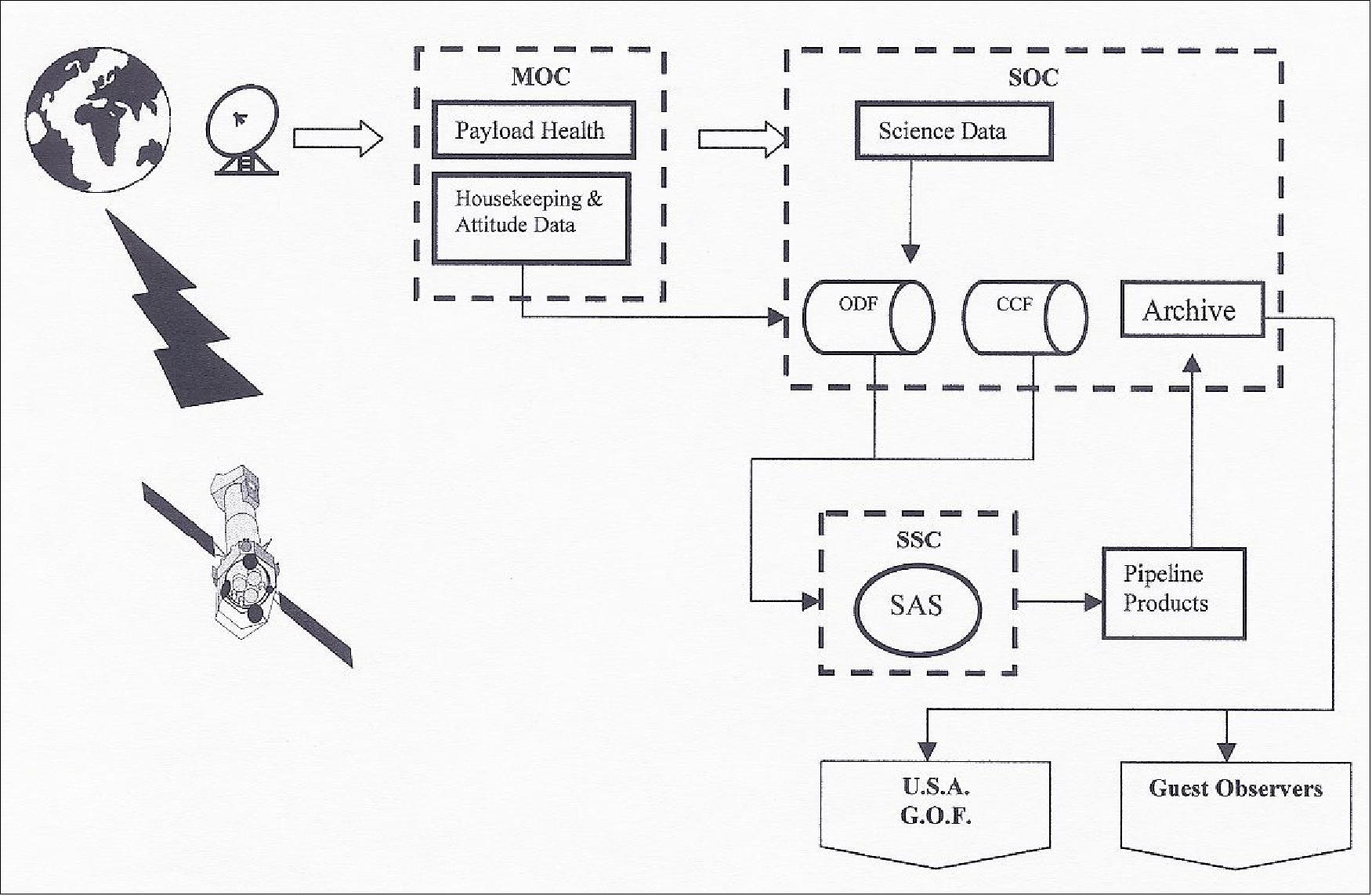
References1) David H. Lumb, Norbert Schartel, Fred A. Jansen, ”XMM-Newton Observatory,” Optical Engineering, Vol. 51, Doi: 10.1117/1.OE.51.1.011009, 2012, URL: https://arxiv.org/ftp/arxiv/papers/1202/1202.1651.pdf
2) F. Jansen, D. Lumb, B. Altieri, J. Clavel, M. Ehle, C. Erd, C. Gabriel, M. Guainazzi, P. Gondoin, R. Much, R. Munoz, M. Santos, N. Schartel, D. Texier, G. Vacanti, ”XMM-Newton observatory - I. The spacecraft and operations,” Astronomy & Astrophysics, Vol. 365, L1{L6 (2001), DOI: 10.1051/0004-6361:20000036, URL: http://www.aanda.org/articles/aa/pdf/2001/01/aaxmm39.pdf
3) http://www.cosmos.esa.int/web/xmm-newton/home
4) Daniel de Chambure, Robert Laine, Kees van Katwijk, ”X-ray telescopes for the ESA XMM spacecraft,” Proceedings of SPIE, Vol. 3444, 'X-Ray Optics, Instruments, and Missions,' 313 (November 19, 1998); doi:10.1117/12.331246, San Diego, CA, USA, July 19, 1998, URL od abstract: http://proceedings.spiedigitallibrary.org/proceeding.aspx?articleid=957566
5) ”XMM-Newton,” NASA, April 2, 2015, URL: http://science.nasa.gov/missions/xmm-newton/
6) ”XMM-Newton Fact Sheet,” ESA, URL: http://sci.esa.int/xmm-newton/47370-fact-sheet/
7) H. Barré, H. Nye, G. Janin, ”An Overview of the XMM Observatory System,” ESA Bulletin No 100, December 1999, URL: http://www.esa.int/esapub/bulletin/bullet100/BARRE.pdf
8) A. Elfving, G. Bagnasco, ”The Pointing and Alignment of XMM,” ESA Bulletin No 100, December 1999, URL: http://www.esa.int/esapub/bulletin/bullet100/ELFVING-BAGNASCO.pdf
9) K. van Katwijk, T. van der Laan, D. Stramaccioni, ”Mechanical and Thermal Design of XMM,” ESA Bulletin, No 100, pp: 30-42, December 1999, URL: http://www.esa.int/esapub/bulletin/bullet100/KATWIJK.pdf
10) D. de Chambure, R. Lainé, K. van Katwijk, P. Kletzkine, ”XMM’s X-Ray Telescopes,” ESA Bulletin, No 100, Dec. 1999, URL: http://www.esa.int/esapub/bulletin/bullet100/CHAMBURE.pdf
11) ”The Telescopes of XMM-Newton,” ESA, URL: http://www.cosmos.esa.int/web/xmm-newton/technical-details-mirrors
12) B. Jackson, ”XMM’s Electrical Power Subsystem,” ESA Bulletin No 100, December 1999, URL: http://www.esa.int/esapub/bulletin/bullet100/JACKSON.pdf
13) A. Karlsson,”XMM’s Data-Handling Subsystem,” ESA Bulletin No 100, December 1999, URL: http://www.esa.int/esapub/bulletin/bullet100/KARLSSON.pdf
14) Ph. Kletzkine, ”The Integration and Testing of XMM,” ESA Bulletin No 100, December 1999, URL: http://www.esa.int/esapub/bulletin/bullet100/KLETZKINE.pdf
15) ”Science@ESA: Episode 5: The untamed, violent Universe,” ESA, 4 July 2013, URL. https://m.esa.int/spaceinvideos/Videos/2013/07/Science_ESA_Episode_5_The_untamed_violent_Universe
16) ”Cosmic manatee accelerates particles from head,” ESA Science & Exploration, 04 July 2022, URL: https://www.esa.int/ESA_Multimedia/Images/2022/07/Cosmic_manatee_accelerates_particles_from_head
17) S. Safi-Harb , B. Mac Intyre, S. Zhang, I. Pope, S. Zhang, N. Saffold, K. Mori, E. V. Gotthelf, F. Aharonian, M. Band, C. Braun, K. Fang, C. Hailey, M. Nynka, and C. D. Rho, ”Hard X-ray emission from the eastern jet of SS 433 powering the W50 ‘Manatee’ nebula: Evidence for particle re-acceleration,” Astrophysical Journal, Draft version July 4, 2022, URL: https://arxiv.org/pdf/2207.00573.pdf
18) ”Could this be a planet in another galaxy?,” ESA Science & Exploration, 25 October 2021, URL: https://www.esa.int/Science_Exploration/Space_Science/Could_this_be_a_planet_in_another_galaxy
19) ”Chandra Sees Evidence for Possible Planet in Another Galaxy,” Harvard Press Release, 26 October 2021, URL: https://chandra.harvard.edu/press/21_releases/press_102521.html
20) A. Wolszczan & D. A. Frail, ”A planetary system around the millisecond pulsar PSR1257 + 12,” Nature, Volume 355, pp: 145-147, Published: 09 January 1992, https://doi.org/10.1038/355145a0
21) Rosanne Di Stefano, Julia Berndtson, Ryan Urquhart, Roberto Soria, Vinay L. Kashyap, Theron W. Carmichael and Nia Imara, ”A possible planet candidate in an external galaxy detected through X-ray transit,” Nature Astronomy , 25 October 2021, URL: https://drive.google.com/file/d/1JSZA4VQD0UxTRUIdnreGX04XVS2I7kUS/view
22) ”XMM-Newton sees light echo from behind a black hole,” ESA Science & Exploration, 28 July 2021, URL: https://www.esa.int/ESA_Multimedia/Images/2021/07/XMM-Newton_sees_light_echo_from_behind_a_black_hole
23) D. R. Wilkins, L. C. Gallo, E. Costantini, W. N. Brandt & R. D. Blandford, ”Light bending and X-ray echoes from behind a supermassive black hole,” Nature, Volume 595, pp: 657-660, Published: 28 July 2021, https://doi.org/10.1038/s41586-021-03667-0
24) ”40-Year Mystery Solved: Source of Jupiter’s X-Ray Flares Uncovered,” NASA/JPL News, 13 July 2021, URL: https://www.jpl.nasa.gov/news/40-year-mystery-solved-source-of-jupiters-x-ray-flares-uncovered?utm_source=iContact&utm_medium=email&utm_campaign=nasajpl&utm_content=juno20210713-1
25) Zhonghua Yao, William R. Dunn, Emma E. Woodfield, George Clark, Barry H. Mauk, Robert W. Ebert, Denis Grodent, Bertrand Bonfond, Dongxiao Pan, I. Jonathan Rae, Binbin Ni, Ruilong Guo, Graziella Branduardi-Raymont, Affelia D. Wibisono, Pedro Rodriguez, Stavros Kotsiaros, Jan-Uwe Ness, Frederic Allegrini, William S. Kurth, G. Randall Gladstone, Ralph Kraft, Ali H. Sulaiman, Harry Manners, Ravindra T. Desai and Scott J. Bolton, ”Revealing the source of Jupiter’s x-ray auroral flares,” Science Advances, Published: 09 July 2021.Vol. 7, No. 28, eabf0851, https://doi.org/10.1126/sciadv.abf0851
26) ”The mystery of what causes Jupiter’s X-ray auroras is solved,” ESA / Science & Exploration / Space Science, 9 July 2021, URL: https://www.esa.int/Science_Exploration/Space_Science/The_mystery_of_what_causes_Jupiter_s_X-ray_auroras_is_solved
27) Binzheng Zhang, Peter A. Delamere, Zhonghua Yao, Bertrand Bonfond, D. Lin, Kareem A. Sorathia, Oliver J. Brambles, William Lotko, Jeff S. Garretson, Viacheslav G. Merkin, Denis Grodent, William R. Dunn and John G. Lyon, ”How Jupiter’s unusual magnetospheric topology structures its aurora,” Science Advances, Vol. 7, No 15, eabd1204, Published: 09 April 2021, https://doi.org/10.1126/sciadv.abd1204
28) ”Orphan cloud discovered in galaxy cluster,” ESA Science & Exploration, 29 June 2021, URL: https://www.esa.int/ESA_Multimedia/Images/2021/06/Orphan_cloud_discovered_in_galaxy_cluster
29) Chong Ge, Rongxin Luo, Ming Sun, Masafumi Yagi, Pavel Jáchym, Alessandro Boselli, Matteo Fossati, Paul E J Nulsen, Craig Sarazin, Tim Edge, Giuseppe Gavazzi, Massimo Gaspari, Jin Koda, Yutaka Komiyama, Michitoshi Yoshida, ”An H α/X-ray orphan cloud as a signpost of intracluster medium clumping,” Monthly Notices of the Royal Astronomical Society, Volume 505, Issue 4, August 2021, Pages 4702–4716, Published: 01 June 2021, , https://doi.org/10.1093/mnras/stab1569
30) Glenn Roberts Jr., ”Study: X-Rays Surrounding ‘Magnificent 7’ May Be Traces of Sought-After Particle,” Berkeley Lab News Release, 15 January 2021, URL: https://newscenter.lbl.gov/2021/01/15/study-x-rays-surrounding-magnificent-7-may-be-traces-of-sought-after-particle/
31) Malte Buschmann, Raymond T. Co, Christopher Dessert, and Benjamin R. Safdi, ”Axion Emission Can Explain a New Hard X-Ray Excess from Nearby Isolated Neutron Stars,” Physical Review Letters, Volume 126, 021102, Published: 12 January 2021, https://doi.org/10.1103/PhysRevLett.126.021102, URL: https://journals.aps.org/prl/pdf/10.1103/PhysRevLett.126.021102
32) ”Cosmic Neon Lights,” ESA Science & Exploration, 11 January 2021, URL: https://www.esa.int/ESA_Multimedia/Images/2021/01/Cosmic_Neon_Lights
33) Lidia M. Oskinova, Vasilii V. Gvaramadze, Götz Gräfener, Norbert Langer and Helge Todt, ”X-rays observations of a super-Chandrasekhar object reveal an ONe and a CO white dwarf merger product embedded in a putative SN Iax remnant,” Astronomy & Astrophysics, Volume 644, Published online: 14 December 2020, https://doi.org/10.1051/0004-6361/202039232
34) ”Cosmic furnace seen by XMM-Newton,” ESA Science & Exploration, 12 November 2020, URL: https://www.esa.int/ESA_Multimedia/Images/2020/11/Cosmic_furnace_seen_by_XMM-Newton
35) ”XMM-Newton spies youngest baby pulsar ever discovered,” ESA Science & Exploration, 17 June 2020, URL: http://www.esa.int/Science_Exploration/Space_Science/XMM-Newton_spies_youngest_baby_pulsar_ever_discovered
36) P. Esposito, N. Rea, A. Borghese, F. Coti Zelati, D. Viganò, G. L. Israel, A. Tiengo, A. Ridolfi, A. Possenti, M. Burgay, D. Götz, F. Pintore, L. Stella, C. Dehman, M. Ronchi, S. Campana, A. Garcia-Garcia, V. Graber, S. Mereghetti, R. Perna, G. A. Rodríguez Castillo, R. Turolla, and S. Zane, ”A Very Young Radio-loud Magnetar,” The Astrophysical Journal Letters, Volume 896, Number 2, Published: 17 June 2020, https://doi.org/10.3847/2041-8213/ab9742
37) ”A bent bridge between two galaxy clusters,” ESA / Science & Exploration / Space Science, 11 May 2020, URL: http://www.esa.int/Science_Exploration/Space_Science/A_bent_bridge_between_two_galaxy_clusters
38) ”Rethinking cosmology: Universe expansion may not be uniform,” ESA / Science & Exploration / Space Science, 8 April 2020, URL: http://www.esa.int/Science_Exploration/Space_Science/Rethinking_cosmology_Universe_expansion_may_not_be_uniform
39) K. Migkas, G. Schellenberger, T. H. Reiprich, F. Pacaud, M. E. Ramos-Ceja and L. Lovisari, ”Probing cosmic isotropy with a new X-ray galaxy cluster sample through the LX–T scaling relation,” Astronomy & Astrophysics, Volume 636, A15, Published online: 8 April 2020, https://doi.org/10.1051/0004-6361/201936602, URL: https://www.aanda.org/articles/aa/pdf/2020/04/aa36602-19.pdf
40) ”The most powerful black hole eruption in the Universe,” ESA / Science & Exploration / Space Science, 27 February 2020, , URL: http://www.esa.int/Science_Exploration/Space_Science/The_most_powerful_black_hole_eruption_in_the_Universe
41) ”XMM-Newton reveals giant flare from a tiny star,” ESA / Science & Exploration / Space Science, 20 February 2020, URL: http://www.esa.int/Science_Exploration/Space_Science/XMM-Newton_reveals_giant_flare_from_a_tiny_star
42) Andrea De Luca, Beate Stelzer, Adam J. Burgasser, Daniele Pizzocaro, Piero Ranalli, Stefanie Raetz, Martino Marelli, Giovanni Novara, Cristian Vignali, Andrea Belfiore, Paolo Esposito, Paolo Franzetti, Marco Fumana, Roberto Gilli, Ruben Salvaterra and Andrea Tiengo, ”EXTraS discovery of an X-ray superflare from an L dwarf,” Astronomy & Astrophysics, Volume 634, February 2020, Article No L13, https://doi.org/10.1051/0004-6361/201937163, URL: https://www.aanda.org/articles/aa/pdf/2020/02/aa37163-19.pdf
43) ”XMM-Newton maps black hole surroundings,” ESA / Science & Exploration / Space Science, 20 January 2020, URL: http://www.esa.int/Science_Exploration/Space_Science/XMM-Newton_maps_black_hole_surroundings
44) William N. Alston, Andrew C. Fabian, Erin Kara, Michael L. Parker, Michal Dovciak, Ciro Pinto, Jiachen Jiang, Matthew J. Middleton, Giovanni Miniutti, Dominic J. Walton, Dan R. Wilkins, Douglas J. K. Buisson, Maria D. Caballero-Garcia, Edward M. Cackett, Barbara De Marco, Luigi C. Gallo, Anne M. Lohfink, Chris S. Reynolds, Phil Uttley, Andrew J. Young & Abderahmen Zogbhi, ”A dynamic black hole corona in an active galaxy through X-ray reverberation mapping,” Nature Astronomy Letter, Published 20 January 2020, https://doi.org/10.1038/s41550-019-1002-x
45) ”The dynamic behavior of a black hole corona,” ESA Science & Exploration, 20 January 2020, URL: http://www.esa.int/ESA_Multimedia/Images/2020/01/The_dynamic_behaviour_of_a_black_hole_corona
46) ”XMM-Newton discovers scorching gas in Milky Way’s halo,” ESA / Science & Exploration / Space Science, 16 January 2020, URL: http://www.esa.int/Science_Exploration/Space_Science/XMM-Newton_discovers_scorching_gas_in_Milky_Way_s_halo
47) Sanskriti Das, Smita Mathur, Fabrizio Nicastro, and Yair Krongold, ”Discovery of a Very Hot Phase of the Milky Way Circumgalactic Medium with Non-solar Abundance Ratios,” The Astrophysical Journal Letters, Volume 882, Number 2, Published: 6 September 2019, https://doi.org/10.3847/2041-8213/ab3b09
48) ”First sighting of hot gas sloshing in galaxy cluster,” ESA / Science & Exploration / Space Science, 10 January 2020, URL: http://www.esa.int/Science_Exploration/Space_Science/First_sighting_of_hot_gas_sloshing_in_galaxy_cluster
49) J. S. Sanders, K. Dennerl, H. R. Russell, D. Eckert, C. Pinto, A. C. Fabian, S. A. Walker, T. Tamura, J. ZuHone and F. Hofmann, ”Measuring bulk flows of the intracluster medium in the Perseus and Coma galaxy clusters using XMM-Newton,” Astronomy & Astrophysics, Volume 633, January 2020, Article No: A42, https://doi.org/10.1051/0004-6361/201936468 , Published online: 10 January 2020
50) ”XMM-Newton’s 20th anniversary in space,” ESA Science & Exploration, 9 December 2019, URL: http://www.esa.int/ESA_Multimedia/Images/2019/12/XMM-Newton_s_20th_anniversary_in_space
51) ”Unexpected periodic flares may shed light on black hole accretion,” ESA Space Science, 11 September 2019, URL: http://www.esa.int/Our_Activities/Space_Science/Unexpected_periodic_flares_may_shed_light_on_black_hole_accretion
52) G. Miniutti, R. D. Saxton, M. Giustini, K. D. Alexander, R. P. Fender, I. Heywood, I. Monageng, M. Coriat, A. K. Tzioumis, A. M. Read, C. Knigge, P. Gandhi, M. L. Pretorius & B. Agís-González, ”Nine-hour X-ray quasi-periodic eruptions from a low-mass black hole galactic nucleus,” Nature, Letter, https://doi.org/10.1038/s41586-019-1556-x, Published: 11 September 2019
53) ”X-raying a galaxy’s stellar remnants,” ESA Space Science Image of the Week, 26 August 2019, URL: http://www.esa.int/spaceinimages/Images/2019/08/X-raying_a_galaxy_s_stellar_remnants
54) L. Ducci, M. Sasaki, F. Haberl and W. Pietsch, ”X-ray source population study of the starburst galaxy M 83 with XMM-Newton,” Astronomy and Astrophysics, Volume 553, May 2013, Article Number: A7, https://doi.org/10.1051/0004-6361/201321035 , URL: https://www.aanda.org/articles/aa/pdf/2013/05/aa21035-13.pdf
55) ”X-ray serendipity,” ESA Space Science Image of the Week, 08 July 2019, URL:
56) ”Storm in the Teacup quasar,” ESA, Space Science Image of the Week: XMM-Newton shows storm still going strong in Teacup quasar, 06 May 2019, URL: http://www.esa.int/spaceinimages/Images/2019/05/Storm_in_the_Teacup_quasar
57) G. B. Lansbury, M. E. Jarvis, C. M. Harrison, D. M. Alexander, A. Del Moro, A. C. Edge, J. R. Mullaney, A. Thomson, ”Storm in a Teacup: X-ray view of an obscured quasar and superbubble,” The Astrophysical Journal Letters, Vol. 856, No 1, 20 March 2018, https://doi.org/10.3847/2041-8213/aab357 , URL: https://iopscience.iop.org/article/10.3847/2041-8213/aab357/pdf
58) ”Black hole activity in massive galaxy M87,” ESA, Our week through the lens:8-12 April 2019, URL: http://m.esa.int/spaceinimages/Images/2019/04/Black_hole_activity_in_massive_galaxy_M87
59) ”Stargazing technology used to spot cancer,” ESA, 8 April 2019, URL: http://m.esa.int/Our_Activities/Telecommunications_Integrated_Applications/Stargazing_technology_used_to_spot_cancer
60) ”Giant ‘chimneys’ vent X-rays from Milky Way’s core,” ESA, 20 March 2019, URL: http://m.esa.int/Our_Activities/Space_Science/Giant_chimneys_vent_X-rays_from_Milky_Way_s_core
61) G. Ponti, F. Hofmann, E. Churazov, M. R. Morris, F. Haberl, K. Nandra, R. Terrier, M. Clavel & A. Goldwurm, ”An X-ray chimney extending hundreds of parsecs above and below the Galactic Centre,” Nature Letter, Volume 567, pp: 347-350, Published: 20 March 2019, https://doi.org/10.1038/s41586-019-1009-6
62) ”What remains of the stars — Past and future generations of stars in NGC 300,” ESA, Space Science Image of the Week, 25 February 2019, URL: http://m.esa.int/spaceinimages/Images/2019/02/Past_and_future_generations_of_stars_in_NGC_300
63) ”Active galaxies point to new physics of cosmic expansion,” ESA, 28 January 2019, URL: https://m.esa.int/Our_Activities/Space_Science/Active_galaxies_point_to_new_physics_of_cosmic_expansion
64) G. Risaliti & E. Lusso, ”Cosmological constraints from the Hubble diagram of quasars at high redshifts,” Nature Astronomy Letter, Published: 28 January 2019, https://doi.org/10.1038/s41550-018-0657-z
65) ”XMM-Newton captures final cries of star shredded by black hole,” ESA, 9 January 2019, URL: http://m.esa.int/Our_Activities/Space_Science/XMM-Newton_captures_final_cries_of_star_shredded_by_black_hole
66) Dheeraj R. Pasham, Ronald A. Remillard, P. Chris Fragile, Alessia Franchini, Nicholas C. Stone, Giuseppe Lodato, Jeroen Homan, Deepto Chakrabarty, Frederick K. Baganoff, James F. Steiner, Eric R. Coughlin, Nishanth R. Pasham, ” Science Report, 09 January 2019: eaar7480, DOI: 10.1126/science.aar7480, URL of abstract: http://science.sciencemag.org/content/early/2019/01/08/science.aar7480
67) ”From gamma rays to X-rays: new method pinpoints previously unnoticed pulsar emission,” ESA Science and Technology, 21 November 2018, URL: http://sci.esa.int/xmm-newton/60950-from-gamma-rays-to-x-rays-new-method-pinpoints-previously-unnoticed-pulsar-emission/
68) Jian Li, Diego F. Torres, Francesco Coti Zelati, Alessandro Papitto, Matthew Kerr, Nanda Rea, ”Theoretically Motivated Search and Detection of Non-thermal Pulsations from PSRs J1747-2958, J2021+3651, and J1826-1256,” The Astrophysical Journal Letters, Volume 868, Number 2, published 21 November 2018, Doi: https://doi.org/10.3847/2041-8213/aae92b
69) ”Extended life for ESA's science missions,” ESA Science & Technology, 14 November 2018, URL: http://sci.esa.int/director-desk/60943-extended-life-for-esas-science-missions/
70) ”Ancient cold front in Perseus,” ESA, Space Science Image of the Week, 29 October 2018, URL: http://m.esa.int/spaceinimages/Images/2018/10/Ancient_cold_front_in_Perseus
71) ”'Cold Front' in the Perseus Cluster,” NASA, 3 April 2018, URL: https://www.nasa.gov/mission_pages/chandra/images/cold-front-in-the-perseus-cluster.html
72) Stephen A. Walker, John ZuHome, Andy Fabian, Jeremy Sanders, ”The split in the ancient cold front in the Perseus cluster,” Nature Astronomy, Vol. 2, pp: 292-296, Feb 26, 2018, DOI: 10.1038/s41550-018-0401-8, URL: https://arxiv.org/pdf/1803.00898.pdf
73) ”Hot X-ray glow from massive cluster of galaxies,” ESA, Space Science Image of the Week, 08 October 2018, URL: http://m.esa.int/spaceinimages/Images/2018/10/Hot_X-ray_glow_from_massive_cluster_of_galaxies
74) ”The 365 galaxy clusters of the XXL Survey – X-ray view,” ESA, XMM-Newton, 04 October 2018, URL: http://sci.esa.int/xmm-newton/60705-the-365-galaxy-clusters-of-the-xxl-survey-x-ray-view/
75) ”Matter falling into a black hole at 30 percent of the speed of light,” Science Daily, 20 September 2018, URL: https://www.sciencedaily.com/releases/2018/09/180920115537.htm
76) K. A. Pounds, C. J. Nixon, A Lobban, A. R. King, ” An ultra-fast inflow in the luminous Seyfert PG1211 143,” Monthly Notices of the Royal Astronomical Society, Volume 481, Issue 2, 1 December 2018,pp: 1832–1838, online publication: 03 September 2018, https://doi.org/10.1093/mnras/sty2359 , URL: https://tinyurl.com/ybg3d9qt
77) ”Students digging into data archive spot mysterious X-ray source,” ESA, 10 August 2018, URL: http://sci.esa.int/xmm-newton/60533-students-digging-into-data-archive-spot-mysterious-x-ray-source/
78) Sandro Mereghetti, Andrea De Luca, David Salvetti, Andrea Belfiore, Martino Marelli, Adamantia Paizis, Michela Rigoselli, Ruben Salvaterra, Lara Sidoli, Andrea Tiengo, ”EXTraS discovery of a peculiar flaring X-ray source in the Galactic globular cluster NGC 6540,” Astronomy & Astrophysics, Volume 616, Article No: A36, DOI: https://doi.org/10.1051/0004-6361/201833086, published online: 10 August 2018
79) ”Flaring source in NGC 6540,” ESA, 10 August 2018, URL: http://sci.esa.int/xmm-newton/60535-flaring-source-in-ngc-6540/
80) ”Digging deeper: First catalogue of X-ray sources in overlapping observations published,” AIP Potsdam, 25 July 2018, URL: http://www.aip.de/en/news/science/digging-deeper-first-catalogue-of-x-ray-sources-in-overlapping-observations-published
81) I. Traulsen, A. D. Schwope, G. Lamer, J. Ballet, F. Carrera, M. Coriat, M. J. Freyberg, L. Michel, C. Motch, S. R. Rosen, N. Webb, M. T. Ceballos, F. Koliopanos, J. Kurpas, M. Page, M. G. Watson, ”The XMM-Newton serendipitous survey. VIII: The first XMM-Newton serendipitous source catalogue from overlapping observations,” Astronomy & Astrophysics, 25 July 2018, URL: https://arxiv.org/pdf/1807.09178.pdf
82) ”The 3XMM-DR7s catalogue of serendipitous sources from overlapping XMM-Newton observations,” URL: http://xmmssc.irap.omp.eu/Catalogue/3XMM-DR7s/3XMM_DR7stack.html
83) ”XMM-Newton finds missing intergalactic material,” ESA, 20 June 2018: http://sci.esa.int/xmm-newton/60427-xmm-newton-finds-missing-intergalactic-material/
84) F. Nicastro, J. Kaastra, Y. Krongold, S. Borgani, E. Branchini, R. Cen, M. Dadina, C. W. Danforth, M. Elvis, F. Fiore, A. Gupta, S. Mathur, D. Mayya, F. Paerels, L. Piro, D. Rosa-Gonzalez, J. Schaye, J. M. Shull, J. Torres-Zafra, N. Wijers, L. Zappacosta, ” Observations of the missing baryons in the warm–hot intergalactic medium,” Nature Letter, Vol. 558, pp: 406-409, published 20 June 2018, DOI: 10.1038/s41586-018-0204-1, URL of abstract: https://www.nature.com/articles/s41586-018-0204-1
85) ”Star shredded by rare breed of black hole,” ESA, 18 June 2018, URL: http://www.esa.int/Our_Activities/Space_Science/Star_shredded_by_rare_breed_of_black_hole
86) Dacheng Lin, Jay Strader, Eleazar R. Carrasco, Dany Page, Aaron J. Romanowsky, Jeroen Homan, Jimmy A. Irwin, Ronald A. Remillard, Olivier Godet, Natalie A. Webb, Holger Baumgardt, Rudy Wijnands, Didier Barret, Pierre-Alain Duc, Jean P. Brodie, Stephen D. J. Gwyn, ”A luminous X-ray outburst from an intermediate-mass black hole in an off-centre star cluster,” Nature Letter, published on 18 June, 2018, DOI: 10.1038/s41550-018-0493-1, URL: https://arxiv.org/pdf/1806.05692.pdf
87) ”Star-circling bubble of gas,” ESA Space Science Image of the Week, 11 June 2018, URL: http://m.esa.int/spaceinimages/Images/2018/06/Star-circling_bubble_of_gas
88) ”Cosmic blast takes rest at last,” ESA, 31 May 2018, URL: http://sci.esa.int/xmm-newton/60376-cosmic-blast-takes-rest-at-last/
89) P. D’Avanzo, S. Campana, O. S. Salafia, G. Ghirlanda, G. Ghisellini, A. Melandri, M. G. Bernardini, M. Branchesi, E. Chassande-Mottin, S. Covino, V. D’Elia, L. Nava, R. Salvaterra, G. Tagliaferri, S. D. Vergani, ”The evolution of the X-ray afterglow emission of GW 170817/ GRB 170817A in XMM-Newton observations,” Astronomy & Astrophysics, Volume 613, 18 May 2018, Article No: L1, https://doi.org/10.1051/0004-6361/201832664
90) ”Where is the Universe’s missing matter?,” ESA, 18 April 2018, URL: http://m.esa.int/Our_Activities/Space_Science/Where_is_the_Universe_s_missing_matter
91) Jiang-Tao Li, Joel N. Bregman, Q. Daniel Wang, Robert A. Crain, Michael E. Anderson, ”Baryon Budget of the Hot Circumgalactic Medium of Massive Spiral Galaxies,” The Astrophysical Journal Letters, Vol. 855, No 2, Published on 12 March 2018, URL of abstract: http://iopscience.iop.org/article/10.3847/2041-8213/aab2af/meta
92) ”Searching galactic haloes for ‘missing’ matter,” ESA, 18.04.2018, URL: https://www.esa.int/spaceinimages/Images/2018/04/Searching_galactic_haloes_for_missing_matter
93) ”Crab Nebula in ultraviolet,” ESA Space Science Image of the Week, 19.03.2018, URL: http://m.esa.int/spaceinimages/Images/2018/03/Crab_Nebula_in_ultraviolet
94) ”XMM-Newton spies first clear X-ray flares from massive stellar lighthouse,” ESA, 26 Feb. 2018, URL: http://sci.esa.int/xmm-newton/60004-xmm-newton-spies-first-clear-x-ray-flares-from-massive-stellar-lighthouse/
95) Ignazio Pillitteri, Scott J. Wolk, Alyssa Goodman, Salvatore Sciortino, ”Smooth X-ray variability from ρ Ophiuchi A+B A strongly magnetized primary B2 star?,” Astronomy & Astrophysics, Volume 567, July 2014, Article No L4, Doi: https://doi.org/10.1051/0004-6361/201424243
96) Ignazio Pillitteri, Scott J. Wolk, Fabio Reale, Lida Oskinova, ”The early B-type star Rho Ophiuchi A is an X-ray lighthouse,” Astronomy & Astrophysics, Volume 602, June 2017, Article No A92, Doi: https://doi.org/10.1051/0004-6361/201630070
97) I. Pillitteri, L. Fossati, N. Castro Rodriguez, L. Oskinova, S. J. Wolk, ”Detection of magnetic field in the B2 star ρ Ophiuchi A with ESO FORS2,” Astronomy & Astrophysics, Volume 610, February 2018, Article No L3, Doi: https://doi.org/10.1051/0004-6361/201732078
98) ”Stellar winds behaving unexpectedly,” ESA, 2 Feb. 2018, URL: http://m.esa.int/Our_Activities/Space_Science/Stellar_winds_behaving_unexpectedly
99) ”Position of HD 5980,” NASA, ESA, 16 Feb. 2007, URL: http://m.esa.int/spaceinimages/Images/2007/02/Position_of_HD_5980
100) ”Deciphering Eta Carinae’s eruptive twin,” ESA, 02 February 2018, URL: https://m.esa.int/spaceinvideos/Videos/2018/02/Deciphering_Eta_Carinae_s_eruptive_twin
101) ”Crescent nebula,” ESA, Space Science Image of the Week, 11 Dec. 2017, URL: http://m.esa.int/spaceinimages/Images/2017/12/Crescent_nebula
102) ”Green light for continued operations of ESA science missions,” ESA, 7 Dec. 2017, URL: http://sci.esa.int/director-desk/59839-green-light-for-continued-operations-of-esa-science-missions/
103) ”Surprisingly erratic X-ray auroras discovered at Jupiter,” ESA Space Science, 30 October 2017, URL: http://www.esa.int/Our_Activities/Space_Science/Surprisingly_erratic_X-ray_auroras_discovered_at_Jupiter
104) W. R. Dunn, G. Branduardi-Raymont, L. C. Ray, C. M. Jackman, R. P. Kraft, R. F. Elsner, I. J. Rae, Z. Yao, M. F. Vogt, G. H. Jones, G. R. Gladstone, G. S. Orton, J. A. Sinclair, P. G. Ford, G. A. Graham, R. Caro-Carretero, A. J. Coates, ”The independent pulsations of Jupiter’s northern and southern X-ray auroras,” Nature Astronomy (2017), doi:10.1038/s41550-017-0262-6, Published online: 30 October 2017
105) ”X-rays Reveal Temperament of Possible Planet-Hosting Stars,” NASA, 6 Sept. 2017, URL: https://www.nasa.gov/mission_pages/chandra/news/x-rays-reveal-temperament-of-possible-planet-hosting-stars.html
106) ”X-rays reveal temperament of possible planet-hosting stars,” Phys.org news, 6 Sept. 2017, URL: https://phys.org/news/2017-09-x-rays-reveal-temperament-planet-hosting-stars.html
107) R. S. Booth, K. Poppenhaeger, C. A. Watson, V. Silva Aguirre, S. J. Wolk, ”An Improved Age-Activity Relationship for Cool Stars older than a Gigayear,” MNRAS (Monthly Notices of the Royal Astronomical Society), 29 June 2017, DOI: 10.1093/mnras/stx1630, URL: https://arxiv.org/pdf/1706.08979.pdf
108) ”A Large Fraction of Rapidly-Growing Supermassive Black Holes Evade Census,” ING web news release, 10 July 2017, URL: http://www.ing.iac.es/PR/press/super.html
109) S. Mateos, F. J. Carrera, X. Barcons, A. Alonso-Herrero, A. Hernán-Caballero, M. Page, C. Ramos Almeida, A. Caccianiga, T. Miyaji, A. Blain, ”Survival of the Obscuring Torus in the Most Powerful Active Galactic Nuclei,” The Astrophysical Journal Letters,Vol. 841,No 2, L18 (6pp), June 1, 2017, URL: http://iopscience.iop.org/article/10.3847/2041-8213/aa7268/pdf
110) ”XMM-Newton slew tracks,” ESA, June 6, 2017, URL: http://m.esa.int/spaceinimages/Images/2017/06/XMM-Newton_slew_tracks
111) ”Sources in XMM-Newton’s second slew catalogue,” ESA Space Science image of the Week , May 15, 2017, URL: http://m.esa.int/spaceinimages/Images/2017/05/Sources_in_XMM-Newton_s_second_slew_catalogue
112) ”Image Release: A New Look at the Crab Nebula,” NRAO (National Radio Astronomy Observatory), May 10, 2017, URL: https://public.nrao.edu/news/image-release-crab-nebula/
113) ”Observatories Combine to Crack Open the Crab Nebula,” NASA, May 10, 2017, URL: https://www.nasa.gov/feature/goddard/2017/observatories-combine-to-crack-open-the-crab-nebula
114) G. Dubner, G. Castelletti, O. Kargaltsev, G. G. Pavlov, M. Bietenholz, A. Talavera, ”Morphological Properties of the Crab Nebula: A Detailed Multiwavelength Study Based on New VLA, HST, Chandra, and XMM-Newton Images,” The Astrophysical Journal, Volume 840, Number 2 , Published May 10, 2017, URL of abstract: http://iopscience.iop.org/article/10.3847/1538-4357/aa6983
115) ”Rapid changes point to origin of ultra-fast black hole winds,” ESA, 01 March, 2017, URL: http://m.esa.int/Our_Activities/Space_Science/Rapid_changes_point_to_origin_of_ultra-fast_black_hole_winds
116) Michael L. Parker, Ciro Pinto, Andrew C. Fabian, Anne Lohfink, Douglas J. K. Buisson, William N. Alston, Erin Kara, Edward M. Cackett, Chia-Ying Chiang, Thomas Dauser, Barbara De Marco, Luigi C. Gallo, Javier Garcia, Fiona A. Harrison, Ashley L. King, Matthew J. Middleton, Jon M. Miller, Giovanni Miniutti, Christopher S. Reynolds, Phil Uttley, Ranjan Vasudevan, Dominic J. Walton, Daniel R. Wilkins, Abderahmen Zoghbi, ”The response of relativistic outflowing gas to the inner accretion disk of a black hole,” Nature Letter, Vol. 543, pp: 83-86, doi:10.1038/nature21385, publish online 01 March, 2017, URL of abstract: http://www.nature.com/nature/journal/v543/n7643/full/nature21385.html
117) ”The brightest, furthest pulsar in the Universe,” ESA, Feb. 21, 2017, URL: http://m.esa.int/Our_Activities/Space_Science/The_brightest_furthest_pulsar_in_the_Universe
118) Gian Luca Israel, Andrea Belfiore, Luigi Stella, Paolo Esposito, Piergiorgio Casella, Andrea De Luca, Martino Marelli, Alessandro Papitto, Matteo Perri, Simonetta Puccetti, Guillermo A. Rodríguez Castillo, David Salvetti, Andrea Tiengo, Luca Zampieri, Daniele D’Agostino, Jochen Greiner, Frank Haber, Giovanni Novara, Ruben Salvaterra, Roberto Turolla, Mike Watson, Joern Wilms, Anna Wolter, ”An accreting pulsar with extreme properties drives an ultraluminous x-ray source in NGC 5907,” Science, 20 Feb 2017, DOI: 10.1126/science.aai8635
119) ”Mind the Gap: Rapid Burster behavior explained,” ESA, Jan. 31, 2017, URL: http://sci.esa.int/xmm-newton/58747-mind-the-gap-rapid-burster-behaviour-explained/
120) J. van den Eijnden, T. Bagnoli, N. Degenaar, A. M. Lohfink, M. L. Parker, J. J. M in ‘t Zand, A. C. Fabian, ”A strongly truncated inner accretion disc in the Rapid Burster,” MNRAS (Monthly Notices of the Royal Astronomical Society) Letters, published on 05 Dec. 2016, Vol. 466, Issue 1, March 2017, DOI: https://doi.org/10.1093/mnrasl/slw244
121) ”Light curve of the Rapid Burster,” ESA, Jan. 31, 2017, URL: http://sci.esa.int/xmm-newton/58749-light-curve-of-the-rapid-burster/
122) ”False-color view of galaxy M81,” ESA, Dec. 19, 2016, URL: http://m.esa.int/spaceinimages/Images/2016/12/False-colour_view_of_galaxy_M81
123) ”Teaching an old satellite new tricks,” ESA, Dec. 8, 2016, URL: http://www.esa.int/Our_Activities/Operations/Teaching_an_old_satellite_new_tricks
124) ”Space selfie,” ESA, Operations image of the week, Dec. 8, 2016, URL: http://www.esa.int/spaceinimages/Images/2016/12/Space_selfie
125) ”Two-year extensions confirmed for ESA's science missions,” ESA, Nov. 22, 2016, URL: http://sci.esa.int/director-desk/58589-two-year-extensions-confirmed-for-esa-s-science-missions/
126) ”XMM-Newton reveals the Milky Way's explosive past,” ESA, Aug. 29, 2016, URL: http://sci.esa.int/xmm-newton/58189-xmm-newton-reveals-the-milky-ways-explosive-past/
127) F. Nicastro, F. Senatore, Y. Krongold, S. Mathur, M. Elvis, ”A Distant Echo of Milky Way Central Activity closes the Galaxy's Baryon Census,” Astrophysics of Galaxies, July 14, 2016, DOI: 10.3847/2041-8205/828/1/L12, URL: https://arxiv.org/pdf/1604.08210v2.pdf
128) ”Milky Way Had a Blowout Bash 6 Million Years Ago,” Harvard-Smithsonian Center for Astrophysics (CFA), Release No 2016-20, Aug. 29, 2016, URL: https://web.archive.org/web/20190511170700/https://www.cfa.harvard.edu/news/2016-20
129) ”Gravitational vortex provides new way to study matter close to a black hole,” ESA, July 12, 2016, URL: http://sci.esa.int/xmm-newton/58072-gravitational-vortex-provides-new-way-to-study-matter-close-to-a-black-hole/
130) Adam Ingram, Michiel van der Klis, Matthew Middleton, Chris Done, Diego Altamirano, Lucy Heil, Phil Uttley, Magnus Axelsson, ”A quasi-periodic modulation of the iron line centroid energy in the black hole binary H1743-322,” MNRAS (Monthly Notices of the Royal Astronomical Society), Volume 461, Issue 2,July 2016, pp: 1967-1980, URL: http://mnras.oxfordjournals.org/content/461/2/1967.full.pdf#page=1&view=FitH
131) Elizabeth Landau, Adam Ingram, Norbert Schartel, ”Black Hole Makes Material Wobble Around It,” NASA/JPL, July 12, 2016, URL: http://www.jpl.nasa.gov/news/news.php?release=2016-184
132) XMM-Newton 2016 Science Workshop - XMM-Newton: The Next Decade, ESAC, Madrid, Spain, 9th - 11th May 2016, URL: http://sci.esa.int/xmm-newton/56848-xmm-newton-2016-science-workshop/
133) Thomas Godard, Wernke zur Borg, Karlie Yeung, Uwe Weissmann, Liviu Toma, Marcus G. F. Kirsch, ”Automated on-ground FDIR for ESA’s XMM-Newton mission,” Proceedings of the 14th International Conference on Space Operations (SpaceOps 2016), Daejeon, Korea, May 16-20, 2016, paper: AIAA 2016 2507, URL: http://arc.aiaa.org/doi/pdf/10.2514/6.2016-2507
134) Uwe Weissmann, Bernd Schuerenberg, Nikolai von Krusenstiern, Marcus G. F. Kirsch, ”In-flight Tank Replenishment of ESA’s XMM-Newton Space Observatory,” Proceedings of the 14th International Conference on Space Operations (SpaceOps 2016), Daejeon, Korea, May 16-20, 2016, paper: AIAA 2016 2308, URL: http://arc.aiaa.org/doi/pdf/10.2514/6.2016-2308
135) ”Powerful winds spotted from mysterious X-ray binaries,” ESA, April 28, 2016, URL: http://sci.esa.int/xmm-newton/57775-powerful-winds-spotted-from-mysterious-x-ray-binaries/
136) Ciro Pinto, Matthew J. Middleton, Andrew C. Fabian, ”Resolved atomic lines reveal outflows in two ultraluminous X-ray sources,” Nature Letter, Vol. 533, pp: 64–67, 05 May 2016, doi:10.1038/nature17417
137) ”Found: Andromeda’s first spinning neutron star,” ESA, March 31, 2016, URL: http://m.esa.int/Our_Activities/Space_Science/Found_Andromeda_s_first_spinning_neutron_star
138) P. Esposito, G. L. Israel, A. Belfiore, G. Novara, L. Sidoli, G. A. Rodrıguez Castillo, A. De Luca, A. Tiengo, F. Haberl, R. Salvaterra, A. M. Read, D. Salvetti, S. Sandrelli, M. Marelli, J. Wilms, D. D’Agostino, “EXTraS discovery of an 1.2-s X-ray pulsar in M31” Monthly Notices of the Royal Astronomical Society, Volume 457, pp L5-L9, Issue 1, March 21, 2016, URL: https://arxiv.org/pdf/1512.00467.pdf
139) ”Unravelling the Cosmic Web: Survey gives insights into Universe's structure,” ESA, Dec. 15, 2015, URL: http://sci.esa.int/xmm-newton/57031-unravelling-the-cosmic-web-survey-gives-insights-into-universes-structure/
140) ”A&A special feature - The XXL Survey: First results,” Astronomy & Astrophysics Press Release, Dec. 15, 2015, URL: http://www.aanda.org/images/stories/PressRelease/2015/xxl/prXXLfinal.pdf
141) ”A new technique to gauge the distant Universe,” ESA, Dec. 3, 2015, URL: http://sci.esa.int/xmm-newton/56983-a-new-technique-to-gauge-the-distant-universe/
142) G. Risaliti, E. Lusso, ”A Hubble Diagram for Quasars,” The Astrophysical Journal, Volume 815, Number 1, Dec. 3, 2015
143) ”The tumultuous heart of our Galaxy,” ESA, Aug.20, 2015, URL:http://m.esa.int/Our_Activities/Space_Science/The_tumultuous_heart_of_our_Galaxy
144) G. Ponti, M. R. Morris, R. Terrier, F. Haberl, R. Sturm, M. Clavel, S. Soldi, A. Goldwurm, P. Predehl, K. Nandra, G. Bélanger, R. S. Warwick, V. Tatischeff, ”The XMM–Newton view of the central degrees of the Milky Way ,” Monthly Notices of the Royal Astronomical Society, October 11, 2015, Vol. 453, doi: 10.1093/mnras/stv1331, pp:172-213, URL: http://mnras.oxfordjournals.org/content/453/1/172.full.pdf#page=1&view=FitH
145) ”Widespread wind from black hole can shape star formation,” ESA, Feb. 19, 2015, URL: http://sci.esa.int/xmm-newton/55475-widespread-wind-from-black-hole-can-shape-star-formation/
146) E. Nardini, J. N. Reeves, J. Gofford, F. A. Harrison, G. Risaliti, V. Braito, M. T. Costa, G. A. Matzeu, D. J. Walton, E. Behar, S. E. Boggs, F. E. Christensen, W. W. Craig, C. J. Hailey, G. Matt, J. M. Miller, P. T. O’Brien, D. Stern, T. J. Turner, M. J. Ward, "Black hole feedback in the luminous quasar PDS 456," Science 20 Feb 2015,Vol. 347, Issue 6224, pp. 860-863, DOI: 10.1126/science.1259202
147) ”XMM-Newton spots monster black hole hidden in tiny galaxy,” ESA, Dec. 19, 2014, URL: http://sci.esa.int/xmm-newton/55114-xmm-newton-spots-monster-black-hole-hidden-in-tiny-galaxy/
148) N. J. Secrest, S. Satyapal, M. Gliozzi, B. Rothberg, S. L. Ellison, W. S. Mowry, J. L. Rosenberg, J. Fischer, H. Schmitt, "An optically obscured AGN in a low mass, irregular dwarf galaxy: A multi-wavelength analysis of J1329+3234," The Astrophysical Journal, Vol. 798, Issue 1, Dec. 18, 2014, URL: http://iopscience.iop.org/article/10.1088/0004-637X/798/1/38/pdf
149) ”Unravelling the Cosmic Web: Survey gives insights into Universe's structure,” ESA, Dec. 15, 2015, URL: http://sci.esa.int/xmm-newton/57031-unravelling-the-cosmic-web-survey-gives-insights-into-universes-structure/
150) ”Working life extensions for ESA’s science missions,” ESA, Nov. 20, 2014, URL: http://sci.esa.int/director-desk/54999-working-life-extensions-for-esas-science-missions/
151) ”Bizarre nearby blast mimics Universe’s most ancient stars,” ESA, July 11, 2014, URL: http://m.esa.int/Our_Activities/Space_Science/Bizarre_nearby_blast_mimics_Universe_s_most_ancient_stars
152) Luigi Piro, Eleonora Troja, Bruce Gendre, Gabriele Ghisellini, Roberto Ricci, Keith Bannister, Fabrizio Fiore, Lauren A. Kidd, Silvia Piranomonte, Mark H. Wieringa, “A Hot Cocoon in the Ultralong GRB 130925A: Hints of a PopIII-like Progenitor in a Low Density Wind Environment,” The Astrophysical Journal Letters, Volume 790, Number 2 , Aug. 1, 2014, URL: http://iopscience.iop.org/article/10.1088/2041-8205/790/2/L15/pdf
153) Marcus G. F. Kirsch, Jim Martin, Andreas Rudolph, Alastair McDonald, Rainer Kresken, Anders Elfving, Mauro Pantaleoni, Thomas Godard, Norbert Pfeil, Timothy Finn, Frederic Schmidt , Detlef Webert, Uwe Weissmann, Andre Vasconcelos, “Extending the lifetime of ESA’s X-ray observatory XMM-Newton,” SpaceOps 2014, 13th International Conference on Space Operations, Pasadena, CA, USA, May 5-9, 2014, paper: AIAA 2014-1608, URL: http://arc.aiaa.org/doi/pdf/10.2514/6.2014-1608
154) ”Unique pair of hidden black holes discovered by XMM-Newton,” ESA, April 22, 2014, URL: http://sci.esa.int/xmm-newton/53980-unique-pair-of-hidden-black-holes-discovered-by-xmm-newton/
155) F. K. Liu, Shuo Li, S. Komossa, ”A milliparsec supermassive black hole binary candidate in the galaxy SDSS J120136.02+300305.5,” The Astrophysical Journal, May 10, 2014, Volume 786, doi:10.1088/0004-637X/786/2/103, URL: http://arxiv.org/pdf/1404.4933v2.pdf
156) ”Chandra and XMM-Newton Provide Direct Measurement of Distant Black Hole's Spin,” Harvard Press release, March 5, 2014, URL: http://chandra.harvard.edu/press/14_releases/press_030514.html
157) ”Black hole boasts heavyweight jets,” ESA, Nov. 13, 2013, URL: http://m.esa.int/Our_Activities/Space_Science/Black_hole_boasts_heavyweight_jets
158) María Díaz Trigo, James C. A. Miller-Jones, Simone Migliari, Jess W. Broderick, Tasso Tzioumis, ”Baryons in the relativistic jets of the stellar-mass black-hole candidate 4U 1630-47,” Nature, Vol. 504, pp: 260–262, 12 December 2013, doi:10.1038/nature12672
159) “Latest XMM-Newton Catalog offers new X-ray Vision,” ESA, July 23, 2013, URL: http://sci.esa.int/xmm-newton/52082-latest-xmm-newton-catalogue-offers-new-x-ray-vision/
160) ”XMM-Newton Overview,” ESA, June 4, 2013, URL: http://www.esa.int/Our_Activities/Space_Science/XMM-Newton_overview
161) ”Speedy black hole holds galaxy’s history,” ESA, Feb. 27, 2013, URL: http://m.esa.int/Our_Activities/Space_Science/Speedy_black_hole_holds_galaxy_s_history
162) G. Risaliti, F. A. Harrison, K. K. Madsen, D. J. Walton, S. E. Boggs, F. E. Christensen, W. W. Craig, B. W. Grefenstette, C. J. Hailey, E. Nardini, Daniel Stern, W. W. Zhang,” A rapidly spinning supermassive black hole at the center of NGC 1365,” Nature, Vol. 494, 28 February 2013, doi:10.1038/nature11938
163) ”Rapidly rotating black hole accreting matter,” Feb. 27, 2013, URL: http://m.esa.int/spaceinimages/Images/2013/02/Rapidly_rotating_black_hole_accreting_matter
164) ”Baffling pulsar leaves astronomers in the dark,” ESA, Jan. 24, 2013, URL: http://sci.esa.int/xmm-newton/51314-baffling-pulsar-leaves-astronomers-in-the-dark/
165) W. Hermsen, J. W. T. Hessels, L. Kuiper, J. van Leeuwen, D. Mitra, J. de Plaa, J. M. Rankin, B. W. Stappers, G. A. E. Wright, R. Basu, A. Alexov, T. Coenen, J.-M. Grießmeier, T. E. Hassall, A. Karastergiou, E. Keane, V. I. Kondratiev, M. Kramer, M. Kuniyoshi, A. Noutsos, M. Serylak, M. Pilia, C. Sobey, P. Weltevrede, K. Zagkouris, A. Asgekar, I. M. Avruch, F. Batejat, M. E. Bell, M. R. Bell, M. J. Bentum, G. Bernardi, P. Best, L. Bîrzan, A. Bonafede, F. Breitling, J. Broderick, M. Brüggen, H. R. Butcher, B. Ciardi, S. Duscha, J. Eislöffel, H. Falcke, R. Fender, C. Ferrari, W. Frieswijk, M. A. Garrett, F. de Gasperin, E. de Geus, A. W. Gunst, G. Heald, M. Hoeft, A. Horneffer, M. Iacobelli, G. Kuper, P. Maat, G. Macario, S. Markoff, J. P. McKean, M. Mevius, J. C. A. Miller-Jones, R. Morganti, H. Munk, E. Orrú, H. Paas, M. Pandey-Pommier, V. N. Pandey, R. Pizzo, A. G. Polatidis, S. Rawlings, W. Reich, H. Röttgering, A. M. M. Scaife, A. Schoenmakers, A. Shulevski, J. Sluman, M. Steinmetz, M. Tagger, Y. Tang, C. Tasse, S. ter Veen, R. Vermeulen, R. H. van de Brink, R. J. van Weeren, R. A. M. J. Wijers, M. W. Wise, O. Wucknitz, S. Yatawatta, P. Zarka, ”Synchronous X-ray and Radio Mode Switches: A Rapid Global Transformation of the Pulsar Magnetosphere,” Science, 25 Jan 2013, Vol. 339, Issue 6118, pp. 436-439, DOI: 10.1126/science.1230960
166) ”EPIC MOS1 Event of Rev. 2382,” ESA, Dec. 18, 2012, URL: http://www.cosmos.esa.int/web/xmm-newton/mos1-ccd3
167) ”Final cry of disrupted star points to site of oblivion,” ESA, Aug. 3, 2012, URL: http://sci.esa.int/xmm-newton/50638-final-cry-of-disrupted-star-points-to-site-of-oblivion/
168) R. C. Reis, J. M. Miller, M. T. Reynolds, K. Gültekin, D. Maitra, A. L. King, T. E. Strohmayer, ”A 200-Second Quasi-Periodicity After the Tidal Disruption of a Star by a Dormant Black Hole,” Science 24 Aug 2012, Vol. 337, Issue 6097, pp. 949-951, DOI: 10.1126/science.1223940
169) ”50 years of X-ray astronomy,” ESA, June 18, 2012, URL: http://www.cosmos.esa.int/web/xmm-newton/50-years-xray-astronomy
170) ”Strangely slow pulsar discovered nestled in young supernova remnant,” ESA, Dec. 20, 2011, URL: http://sci.esa.int/xmm-newton/49784-strangely-slow-pulsar-discovered-nestled-in-young-supernova-remnant/
171) V. Hénault-Brunet, L. M. Oskinova, M. A. Guerrero, W. Sun4, Y.-H. Chu, C. J. Evans, J. S. Gallagher III, R. A. Gruendl, J. Reyes-Iturbide, ”Discovery of a Be/X-ray pulsar binary and associated supernova remnant in the Wing of the Small Magellanic Cloud,” Monthly Notices of the Royal Astronomical Society: Letters, Volume 420, Issue 1, pages L13–L17, February 2012, DOI: 10.1111/j.1745-3933.2011.01183.x
172) ”Neutron star caught feasting on clump of stellar matter,” ESA, June 28, 2011, URL: http://sci.esa.int/xmm-newton/48733-neutron-star-caught-feasting-on-clump-of-stellar-matter/
173) E. Bozzo, A. Giunta, G. Cusumano, C. Ferrigno, R. Walter, S. Campana, M. Falanga, G. Israel, L. Stella, ”XMM-Newton observations of IGR J18410-0535: the ingestion of a clump by a supergiant fast X-ray transient,” Astronomy & Astrophysics, Volume 531, July 2011, URL: http://www.aanda.org/articles/aa/pdf/2011/07/aa16726-11.pdf
174) ”An old galaxy cluster discovered in the young Universe,” ESA, March 9, 2011, URL: http://sci.esa.int/xmm-newton/48483-an-old-galaxy-cluster-discovered-in-the-young-universe/
175) R. Gobat, E. Daddi, M. Onodera, A. Finoguenov, A. Renzini, N. Arimoto, R. Bouwens, M. Brusa, R.-R. Chary, A. Cimatti, M. Dickinson, X. Kong, M. Mignoli, ”A mature cluster with X-ray emission at z = 2.07,” Astronomy & Astrophysics, Volume 526, February 2011, URL: http://www.aanda.org/articles/aa/pdf/2011/02/aa16084-10.pdf
176) M. Pierre, F. Pacaud, J. B. Juin, J. B. Melin, P. Valageas, N. Clerc, P. S. Corasaniti,”Precision cosmology with a wide area XMM cluster survey,” Monthly Notes of the Royal Astronomical Society, July 7, 2011, URL: http://arxiv.org/pdf/1009.3182v2.pdf
177) ”XXL: The Ultimate XMM Extragalactic Survey,” ESA. May 2, 2011, URL: http://sci.esa.int/xmm-newton/48357-xxl-the-ultimate-xmm-extragalactic-survey/
178) ”Are most pulsars really magnetars in disguise?,” ESA Oct. 14, 2010, URL: http://sci.esa.int/xmm-newton/47844-are-most-pulsars-really-magnetars-in-disguise/
179) N. Rea, P. Esposito, R. Turolla, G. L. Israel, S. Zane, L. Stella, S. Mereghetti, A. Tiengo, D. Götz, E. Göğüş, C. Kouveliotou, ”A Low-Magnetic-Field Soft Gamma Repeater,” Science 12 Nov 2010, Vol. 330, Issue 6006, pp. 944-946, DOI: 10.1126/science.1196088
180) ”XMM-Newton line detection provides new tool to probe extreme gravity,” ESA, June 21, 2010, URL: http://sci.esa.int/xmm-newton/47225-xmm-newton-line-detection-provides-new-tool-to-probe-extreme-gravity/
181) ”Novel observing mode on XMM-Newton opens new perspectives on galaxy clusters,” ESA, May 31, 2010, URL: http://sci.esa.int/xmm-newton/47086-xmm-newton-opens-new-perspectives-on-galaxy-clusters/
182) ”XMM-Newton releases new edition of cosmic catalog,” ESA, April 28, 2010, URL: http://sci.esa.int/xmm-newton/46958-xmm-newton-releases-new-edition-of-cosmic-catalogue/
183) XMM-Newton 10 years,” ESA, 01 March 20010, URL: https://www.esa.int/spaceinvideos/Videos/2010/03/XMM_10_years
184) ”XMM-Newton traces dark matter in faint, distant galaxy groups,” ESA, Jan. 20, 2010, URL: http://sci.esa.int/xmm-newton/46322-xmm-newton-traces-dark-matter-in-faint-distant-galaxy-groups/
185) ”XMM-Newton celebrates a golden decade of X-ray astrophysics,” ESA, Dec. 10, 2009, URL: http://sci.esa.int/xmm-newton/46071-xmm-newton-s-golden-decade/
186) ”Airbus Defence and Space-built XMM-Newton telescope with sensational results on exploding stars, black holes and galaxy clusters,” Airbus DS, Dec. 3, 2009, URL: http://www.space-airbusds.com/en/press_centre/airbus-defence-and-space-built-xmm-newton-telescope-with-sensational-results-on-exploding-stars-k81.html
187) ”XMM-Newton discovers a new class of black holes,” ESA, July 1, 2009, URL: http://m.esa.int/Our_Activities/Space_Science/XMM-Newton_discovers_a_new_class_of_black_holes
188) ”XMM-Newton and Integral clues on magnetic powerhouses,” ESA, Nov. 14, 2008, URL: http://m.esa.int/Our_Activities/Space_Science/XMM-Newton_and_Integral_clues_on_magnetic_powerhouses
189) ”XMM-Newton talks again loud and clear,” ESA, Oct. 23, 2008, URL: http://m.esa.int/Our_Activities/Space_Science/XMM-Newton_talks_again_loud_and_clear
190) ”XMM-Newton’s massive discovery,” ESA, Aug. 25, 2008, URL: http://m.esa.int/Our_Activities/Space_Science/XMM-Newton_s_massive_discovery
191) ”XMM-Newton watches lazy pulsar being jazzed up by companion,” ESA, June 23, 2008, URL: http://m.esa.int/Our_Activities/Space_Science/XMM-Newton_watches_lazy_pulsar_being_jazzed_up_by_companion
192) A. Pellizzoni, A. Tiengo, A. De Luca, P. Esposito, S. Mereghetti, “PSRJ0737-3039: Interacting pulsars in X-rays,” Astrophysical Journal, May 2008; Preprint of March 28, 2008, URL: http://arxiv.org/pdf/0802.0350.pdf
193) ”XMM-Newton: pulsed heartbeat of a weird new type of star,” ESA, Dec. 21 2007, URL http://m.esa.int/Our_Activities/Space_Science/XMM-Newton_pulsed_heartbeat_of_a_weird_new_type_of_star
194) ”Extension of ESA’s Integral and XMM-Newton missions approved,” ESA, Nov. 14, 2007, URL: http://m.esa.int/Our_Activities/Space_Science/Extension_of_ESA_s_Integral_and_XMM-Newton_missions_approved
195) ”XMM-Newton releases the largest catalogue of X-ray sources,” ESA, Sept. 7, 2007, URL: http://m.esa.int/Our_Activities/Space_Science/XMM-Newton_releases_the_largest_catalogue_of_X-ray_sources
196) http://m.esa.int/spaceinimages/Images/2007/09/2XMM_compared_to_exisiting_catalogues
197) http://www.esa.int/esaSC/SEMAKQRHKHF_index_0.html
198) ”Mapping the ingredients of an exploded star,” ESA, Nov. 27, 2001, URL: http://sci.esa.int/xmm-newton/29047-mapping-the-ingredients-of-an-exploded-star/
199) http://sci.esa.int/jump.cfm?oid=29048
200) ”INFO 03-2000: Eye-openers from XMM-Newton,” ESA, Feb. 9, 2000, URL: http://sci.esa.int/xmm-newton/13201-info-03-2000-eye-openers-from-xmm-newton/
201) ”30 Doradus in LMC,” ESA, Jan. 30, 2000, URL: http://sci.esa.int/xmm-newton/13202-30-doradus-in-lmc/
202) ”Hickson Galaxy Group (HCG16), ESA, Jan. 30, 2000, ” URL: http://sci.esa.int/xmm-newton/13203-hickson-galaxy-group-hcg16/
203) ”Nearby star HR1099,” ESA, Jan. 30, 2000, URL: http://sci.esa.int/xmm-newton/13205-nearby-star-hr1099/
204) ”XMM Newton: First Pictures Announcement,” ESA, 04 February, 2000, URL: https://www.esa.int/spaceinvideos/Videos/2000/02/XMM_Newton_First_Pictures_Announcement
205) M. J. L. Turner, A. Abbey, M. Arnaud, M. Balasini, M. Barbera, E. Belsole, P. J. Bennie, J. P. Bernard, G. F. Bignam, M. Boer, U. Briel, I. Butler, C. Cara, C. Chabaud, R. Cole, A. Collura, M. Conte, A. Cros, M. Denby1, P. Dhez, G. Di Coco, J. Dowson, P. Ferrando, S. Ghizzardi, F. Gianotti, C. V. Goodall, L. Gretton, R. G. Griffiths, O. Hainaut, J. F. Hochedez, A. D. Holland, E. Jourdain, E. Kendziorra, A. Lagostina, R. Laine, N. La Palombara, M. Lortholary, D. Lumb, P. Marty, S. Molendi, C. Pigot, E. Poindron, K. A. Pounds, J. N. Reeves, C. Reppin, R. Rothenflug, P. Salvetat, J. L. Sauvageot, D. Schmitt, S. Sembay, A. D. T. Short, J. Spragg, J. Stephen, L. Strüder, A. Tiengo, M. Trifoglio, J. Trümper, S. Vercellone, L. Vigroux, G. Villa, M. J. Ward, S. Whitehead, E. Zonca, ”The European Photon Imaging Camera on XMM-Newton:The MOS cameras,” Astronomy & Astrophysics, Vol. 365, L27{L35 (2001) DOI: 10.1051/0004-6361:20000087, URL: http://www.aanda.org/articles/aa/pdf/2001/01/aaxmm47.pdf
206) ”The European Photon Imaging Camera (EPIC) onboard XMM-Newton,” ESA, URL: http://www.cosmos.esa.int/web/xmm-newton/technical-details-epic
207) L. Strüder, U. Briel, K. Dennerl, R. Hartmann, E. Kendziorra, N. Meidinger, E. Pfeffermann, C. Reppin, B. Aschenbach, W. Bornemann, H. Bräuninger, W. Burkert, M. Elender, M. Freyberg, F. Haberl, G. Hartner, F. Heuschmann, H. Hippmann, E. Kastelic, S. Kemmer, G. Kettenring, W. Kink, N. Krause, S. Müller, A. Oppitz, W. Pietsch, M. Popp, P. Predehl, A. Read, K. H. Stephan, D. Stötter, J. Trümper, P. Holl, J. Kemmer, H. Soltau, R. Stötter, U. Weber, U. Weichert, C. von Zanthier, D. Carathanassis, G. Lutz, R. H. Richter, P. Solc, H. Böttcher, M. Kuster, R. Staubert, A. Abbey, A. Holland, M. Turner, M. Balasini, G. F. Bignami, N. La Palombara, G. Villa, W. Buttler, F. Gianini, R. Lainé, D. Lumb, P. Dhez, ”The European Photon Imaging Camera on XMM-Newton: The pn-CCD camera,” Astronomy & Astrophysics, Volume 365, Number 1, January I 2001, DOI: 10.1051/0004-6361:20000066, pp: L18 - L26, URL: http://www.aanda.org/articles/aa/pdf/2001/01/aaxmm35.pdf
208) M. G. Watson, J.-L. Augueres, J. Ballet, X. Barcons, D. Barret, M. Boer, Th. Boller, G. E. Bromage, H. Brunner, F. J. Carrera, M. S. Cropper, M. Denby, M. Ehle, M. Elvis, A. C. Fabian, M. J. Freyberg, P. Guillout, J.-M. Hameury, G. Hasinger, D. A. Hinshaw, T. Maccacaro, K. O. Mason, R. G. McMahon, L. Michel, L. Mirioni, J. P. Mittaz, C. Motch, J.-F. Olive, J. P. Osborne, C. G. Page, M. Pakull, B. H. Perry, M. Pierre, W. Pietsch, J. P. Pye, A. M. Read, T. P. Roberts, S. R. Rosen, J.-L. Sauvageot, A. D. Schwope, K. Sekiguchi, G. C. Stewart, I. Stewart, I. Valtchanov, M. J. Ward, R. S. Warwick, R. G.West, N. E.White, D. M. Worrall, ”The XMM-Newton Serendipitous Survey — The role of XMM-Newton Survey Science Center,” Astronomy & Astrophysics, L51{L59 (2001), DOI: 10.1051/0004-6361:20000067, URL: http://xmmssc-www.star.le.ac.uk/XID/XID1watson01.pdf
The information compiled and edited in this article was provided by Herbert J. Kramer from his documentation of: ”Observation of the Earth and Its Environment: Survey of Missions and Sensors” (Springer Verlag) as well as many other sources after the publication of the 4th edition in 2002. - Comments and corrections to this article are always welcome for further updates (eoportal@symbios.space).
Spacecraft Launch Mission Status Sensor Complement Ground Segment References Back to top